5 Planning Phase Two: Assess Site
5.1 Introduction
Phase Two identifies site factors that will limit revegetation establishment, defines the possible mitigating measures that can be employed to reduce the effects of limiting conditions on plant growth, and surveys those site attributes that can be used as resources to accomplish revegetation objectives. By the end of Phase Two, you should have:
- Identified limiting factors,
- Considered mitigating measures for limiting factors, and
- Assessed site resources.
5.1.1 Limiting Factors
Site conditions that affect plant establishment and growth are referred to as limiting factors. Odum (1971) defines limiting factors as "any condition which approaches or exceeds the limits and tolerance (of a plant species)." He further states that "the chief value of the concept of limiting factors lies in the fact that it gives the ecologist an 'entering wedge' into the study of complex situations. Environmental relations of organisms are apt to be complex, so that it is fortunate that not all possible factors are of equal importance in a given situation or for a given organism." For the revegetation specialist, defining the limiting factors on any particular project or site is an essential process in developing a revegetation plan because it identifies from a multitude of site factors only those that are roadblocks to successful revegetation. Not only does this simplify a complex analysis, it requires the revegetation specialist to systematically consider all site factors, focusing on those of greatest concern. For example, typical revegetation treatments conventionally call for the blanket use of fertilizers without assessing if nutrients are really limiting to plant growth. In many cases, other limiting factors to revegetation, such as low rainfall, compacted soils, low organic matter, and poor rooting depth, are more limiting. Without a comprehensive assessment of limiting factors, the prescription for fertilizer is akin to a physician prescribing medicine before the patient has been properly diagnosed. While soil fertility is often important on many highly disturbed sites, it might not be the primary limiting factor to revegetation on the site.
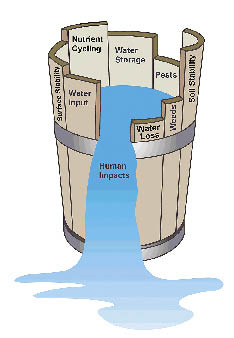
Figure 5.1 — Limiting factors to revegetation can be displayed as unequal boards of a barrel. Water can only be held to the level of most limiting factor.
This manual has grouped the site characteristics essential for plant growth into nine limiting factors to revegetation typically encountered in the western United States. These factors are further broken down into component parts, or parameters (Figure 5.2). In this chapter each limiting factor to revegetation and corresponding parameters are discussed in terms of why they are important to plant establishment and growth, how they are assessed, and what mitigating measures can be applied to make them less limiting.
The information used in defining limiting factors for each revegetation unit can be obtained from the surveys and reports conducted in Phase One. In this process, it is important that an assessment of every limiting factor and corresponding parameter be made for each revegetation unit based on the expected condition of the site after disturbance. For this assessment, the types of post-construction disturbance must be clearly understood.
Mitigating measures are the site treatments that will reduce or eliminate the site conditions limiting to revegetation. There are usually several ways to mitigate each limiting factor. In this phase, mitigating measures are identified and briefly described for each limiting plant factor and parameter. While some of the mitigating measures might seem impractical for a particular revegetation project, they nevertheless should be considered in this part of the assessment. A process for developing the most appropriate set of mitigating measures into an integrated revegetation strategy will be covered in Phase Four.
Figure 5.2 — This manual recognizes nine site characteristics that can limit plant growth. These factors are further broken down into component parts, or parameters.
Critical Plant Factor![]() |
Parameters |
---|---|
1. Water Input | Precipitation, Interception, Infiltration, Road Drainage |
2. Water Storage and Accessibility | Soil Texture, Rock Fragments, Soil Structure, Rooting Depth, Mycorrhizal Fungi |
3. Water Loss | Wind Aspect, Competing Vegetation, Soil Cover |
4. Nutrient Cycling | Topsoil, Site Organic Matter, Nitrogen and Carbon, Nutrients, pH and Salts |
5. Surface Stability | Rainfall and Wind, Freeze-Thaw, Soil Cover, Surface Strength, Infiltration, Slope Gradient, Surface Roughness, Slope Length |
6. Slope Stability | Permeability, Restrictive Layer, Water Input, Slope Length, Slope Gradient, Soil Strength |
7. Weeds | Weed Sources, Weed Growing Environment |
8. Pests | Mammals, Insects |
9. Human Interface | Disease, Road Maintenance, Recreational Use |
5.2 Water Input
Water input refers to the moisture supplied to the soil through rainfall, snowmelt, and road drainage. This moisture recharges the soil and becomes the primary source of water for plant establishment and growth. Water input is influenced by obstacles that capture, or intercept, water before it can enter the soil, including standing live or dead vegetation and soil cover (litter, duff, and mulch). Surface infiltration rates also regulate entry of surface water. If infiltration rates are low, water that would normally enter the soil runs off the surface and is unavailable.
The primary site factors that affect water input are:
- Precipitation,
- Interception,
- Infiltration, and
- Road drainage.
In the western United States, water input is at its lowest levels from late spring through early fall. This is also the period when plants require the most soil moisture for survival and growth. During the summer, when water input is low, the soil profile dries out as vegetation withdraws moisture. As soil moisture is depleted, plants cease growing; if soil moisture is not recharged, plants will go into dormancy or die. It is critical that any water from precipitation arriving during the dry season enters the soil and is stored for later plant use.
5.2.1 Precipitation
In wildlands revegetation, the only source of soil water comes through precipitation in the form of rainfall or snowmelt. In the western United States, this typically occurs from late fall through mid spring, during a period when plants are dormant and least able to utilize soil moisture for growth. Water that is not stored in the soil during these events is lost from the site either to ground water or runoff. The period when plants need soil moisture the most occurs during a five to six month period, from April through October. For most sites in the western United States, the amount of moisture that occurs in this period is less than a quarter of the total annual rainfall.
Vegetation native to the western United States has evolved to compensate for the limited supply of moisture during the growing season (Figure 5.3). During the spring, when soils are charged with moisture from the winter precipitation and soil temperatures increase, plants produce new roots, followed by new foliage. As the soil dries out and plants undergo mild moisture stresses, new root and foliage growth cease. During the summer months, soil continues to dry and plants respond to even greater moisture stress by shutting down their physiological functions and becoming dormant. By mid to late summer, when available soil moisture is depleted and evapotranspiration rates are high, plants will show stress symptoms (browning, loss of needles and leaves); under extreme circumstances, plants will die. By late summer and early fall, rain returns and the soil slowly moistens again, reducing plant moisture stress and signaling plants to grow new roots.
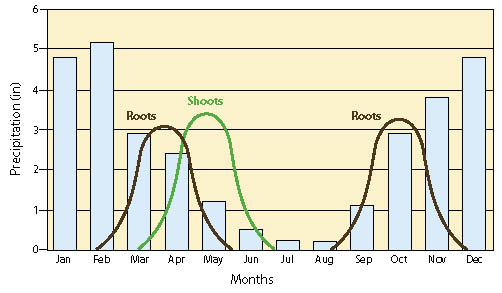
Figure 5.3 — In the western United States, root and shoot growth occur when moisture is available in the spring. Growth ceases by early summer when there is very little rainfall. Root growth takes place again from late September through November when soils are recharged by fall rainstorms.
The primary characteristic of precipitation for plant survival is the quantity of rainfall delivered in each storm event during the dry season. Storm events that deliver more than 0.25 inches of rainfall can wet the surface portion of the soil profile and reduce plant moisture stress. Precipitation events that deliver less than this amount will rarely supply enough water to enter the soil, especially if interception and runoff rates are high.
5.2.1.1 Precipitation — How to Assess
Average monthly rainfall can be estimated by extrapolating climate data from weather stations closest to the project site (See Section 4.3.2 for how to access weather information). For more site specific information, precipitation can be collected on-site using rain gauges that capture and record precipitation.
There are two types of precipitation gauges available — digital and non-digital. The advantages of digital gauges are that they record the amount of rainfall and time that it occurred; the downside is cost (although prices are coming down). There are many types of digital rain gauges available, ranging in price and quality. It is important to select a digital rain gauge that is rugged, self-maintaining, and can record for long periods of time.
Non-digital rain gauges are basically cylinders that collect and store precipitation while preventing evaporation. The gauges are monitored by simply measuring the water in the cylinder. The disadvantage of non-digital rain gauges is that they only report the rainfall that has occurred between site visits. They do not provide the dates when rainfall occurred and do not record rainfall intensities.
5.2.1.2 Mitigating for Low Precipitation
Making the most of rain and snowmelt is an important part of successful revegetation planning. In most cases, supplemental watering will not be feasible. However, if very little water input occurs during the summer months, supplemental water on a temporary basis might be considered during plant establishment. This can take an active form, such as irrigation, or a passive form, such as redirecting surface water to planted seedlings.
Irrigation — Irrigation can be expensive, and it is generally used only on projects with high visibility or when rapid establishment is necessary for slope stability. These are projects where revegetation objectives include minimizing the risk of seedling failure or enhancing vegetation growth.
There are several basic types of irrigation systems used in roadside revegetation. They are grouped into fixed systems, such as overhead sprinkler and drip irrigation, and manually applied systems. Fixed systems are discussed in Section 10.4.5.2, Drip Irrigation. Manual systems require water to be delivered directly to each plant, either from a hose or water container.
If only a few applications are necessary, the entire project can be done by hand. Personnel can water each seedling or seeded area using a water truck or hydroseeder (with water only), although care must be taken to avoid pulling hoses over establishing plants. Creating basins around seedlings will pond the surface-applied water and keep it concentrated in the seedling root zone. However, a better way to be certain that water will be delivered directly to the roots is to integrate the deep pot irrigation system into drip or manually applied irrigation methods (Bainbridge and others 2001). Pipes made from PVC or other materials are placed at depths of 1 to 2 feet beside the seedling at the time of planting. The pipes are then filled with water when the soils dry out in the summer. The advantage of deep pipe irrigation is that water is delivered directly to the root system and, because the water is placed deeper in the soil, roots are forced to extend further into the soil for moisture. Refer to Section 10.4.5.1, Deep Pot Irrigation, for how to install this system.
For any irrigation method, it is important to monitor the wetting pattern of each irrigation. This will assure that water is applied at the appropriate rates. Digging a hole where the water has been applied at least one hour after irrigation will show how far the water has moved into the soil profile. Duration of irrigations can be adjusted accordingly.
Water Harvesting — Water harvesting is the alteration of local topography to capture runoff water and concentrate it in areas where it can be used by plants. Water harvesting designs can be applied to roadside revegetation in several ways. They include, but are not limited to, contour bench terraces, runoff strips, and fill slope microcatchments. Contour bench terraces are structures carved out of cut and fill slopes that collect and store runoff water. When filled with topsoil or amended soil, they are referred to as planting pockets. Figure 5.4 shows how planting pockets collect water. Fill slope microcatchments take advantage of water that drains off road surfaces and shoulders during intense rainstorms by capturing runoff in berms or depressions created at the base of the road shoulder (Figure 5.5). Shrubs and trees planted in these catchment areas will receive greater soil moisture. Even very low rainfall events, which would normally be of insufficient quantity to moisten the soil surface, can recharge soil in planting pockets and fill slope microcatchments. Sediments will also be deposited on the benches and pockets during rainstorms, building the soil up over time and reducing soil erosion.
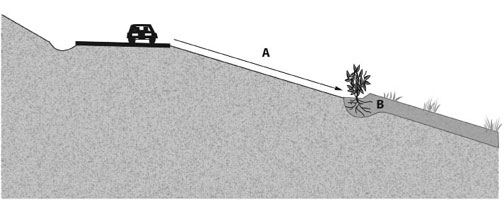
Figure 5.4 — Fill slope microcatchments take advantage of the low infiltration rates of compacted fill slopes. Water moves off impervious road surfaces and compacted road shoulders during rainstorms (A), and is captured in berms or flattened areas below the road shoulder (B). If this area is ripped and amended with organic matter, it becomes a very good environment for establishing shrubs and trees. Berms and/or flattened areas are also catchments for sediments.
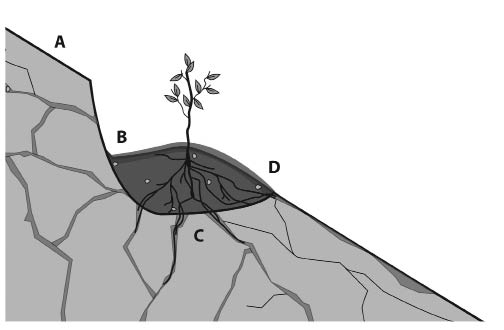
Figure 5.5 — Planting pockets are designed to capture water from upslope runoff (A), that collects in a slight depression (B). Captured water wets up the soil after each rainstorm and drains into the fractured bedrock (C). Soil is protected from surface erosion on the downhill side of the pocket with mulch or erosion fabric (D).
5.2.2 Rainfall Interception
The amount of water entering the soil profile from a rainfall event can be significantly reduced by the interception of live or dead vegetation cover. Rainfall is captured through a series of layers, beginning with 1) the tree and shrub canopy, 2) ground cover, 3) litter, and 4) duff, and is returned to the atmosphere through evaporation. During the dry season, moisture from a low rainfall event might not reach to soil.
5.2.2.1 Rainfall Interception — How to Assess
Interception can be determined by the soil cover and vegetation that exist on the site after construction. In most cases, there will be very little vegetation and ground cover. It is therefore important to understand the effects of various types of ground cover used in revegetation on the rainfall interception. The depth and water-holding capacity of the material will determine the effect on water input.
Water-holding capacity of a material can be measured through testing labs specializing in composts. Alternatively, it can be measured by collecting the soil layer (duff, litter, mulch) and drying this material at 230 °F in an oven or crockpot with a meat thermometer stuck in the material. When the sample is dry (reaching 230 °F), it is placed in a 5-inch long by 3-inch round PVC pipe with the bottom secured with a flat piece of cardboard to prevent the material from falling out. The PVC pipe is weighed and recorded, then placed in a bucket. The bucket is filled with water to the top of the pipe. The sample is removed and tipped upside down on a stack of paper towels to allow the water to drain. Wet towels are exchanged with dry towels until towels are no longer wet. The pipe containing the material is reweighed. The material is then removed and the pipe plus bottom material is weighed. The moisture holding capacity of the material (by % dry weight) is: (wet weight - container) - (dry weight - container))/(dry weight - container)*100.
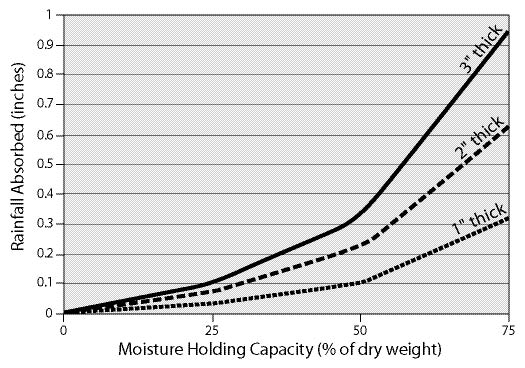
Figure 5.6 — The amount of rainfall intercepted by soil cover (e.g., mulch or litter) is dependent on its water-holding capacity and thickness.
Figure 5.6 can be used to approximate how much rainfall is intercepted based on these parameters.
5.2.2.2 Mitigation for High Rainfall Interception
It is important to consider the water-holding capacities of the mulches to be used, especially on arid sites. Highly decomposed, fine textured composts have high water-holding capacities compared to coarser textured, less decomposed organic materials, such as bark, wood chips, and wood strands. Coarser materials allow more water to reach the soil.
5.2.3 Infiltration
Infiltration is the ability of the soil surface to absorb water from rainfall, snowmelt, irrigation, or road drainage. When infiltration rates are slower than the amount of water applied to the surface of the soil, runoff will occur and this water will not be available for plant uptake. In addition, runoff can detach and transport soil, causing soil erosion and water quality problems. See Section 5.6.5 for a discussion of infiltration rates on surface stability.
The size, abundance, and stability of soil aggregates in the surface soil determine the infiltration rates. Large stable pores created by worms, insects, and root channels will absorb water quickly and have high infiltration rates; soils that have been compacted, topsoil removed, or are low in organic matter will have poor infiltration rates.
Under undisturbed conditions, infiltration rates are typically high, especially where a litter and duff cover exists. When soil cover is removed, the impact from rainsplash can seal the soil surface, creating a crust that will significantly reduce infiltration rates. Infiltration rates are also reduced when the soil is compacted by heavy equipment or traffic.
5.2.3.1 Infiltration — How to Assess
The most accurate method to measure field infiltration rates is using the rainfall simulator (See Figure 5.48). This equipment is calibrated to simulate the appropriate drop size and impact velocity of many rainfall events (Grismer and Hogan 2004). The rainfall simulator is expensive to operate and is not routinely used by the revegetation specialist. The most common application for this technology is in comparing different mitigating measures, such as mulches and tillage methods, on infiltration capacity.
Without conducting rainfall simulation tests, infiltration rates must be inferred by measuring soil strength, using a soil penetrometer, bulk density measurements (See Section 5.3.3), and from site characteristics such as visual observation of compaction and the percentage of soil cover. For most construction activities that remove surface cover or disturb the topsoil, it can be assumed that infiltration rates will be reduced to levels that will create overland flow under most rainfall intensities.
5.2.3.2 Mitigating for Low Infiltration Rates
Minimize Compaction — Driving heavy equipment over soils causes compaction and reduces infiltration rates. After sites have been prepared for seeding or planting, heavy equipment must not be driven over soils. Such practices that are often recommended for erosion control, such as trackwalking, can actually decrease infiltration rates and adversely affect the establishment and cover of native plants. These practices may not be appropriate on all soil types and should be assessed on a site specific basis (Hogan and Grismer 2007).
Tillage — Infiltration rates can be increased through soil tillage, including subsoiling, ripping, and disking (See Section 10.1.2, Tillage). Tillage will, in most cases, reduce compaction and increase macro-pore space in the surface soil, as well as create surface roughness that further increases infiltration rates. Depending on the stability of the surface material and the level of organic matter, the effects of tillage on infiltration might only be effective for a short time. Concentrated water from road drainage has the potential to create deep gullies and must be avoided on tilled soils.
Organic Amendments — Incorporating organic amendments into the soil surface can create large, stable pores. However, unless the pores are interconnecting, they will not drain well (Claassen 2006). One method for creating continuous pores is to use long, slender organic material, like shredded bark or wood, composted yard waste, straw or hay (See Section 10.1.5, Organic Matter Amendments). Compared to short organic materials like wood chips, longer materials can increase infiltration rates. Incorporating higher quantities of organic matter in the soil will also increase porosity because of the potential of the organic material to overlap and interconnect.
Mulch and Tillage — Applying mulch by itself does not necessarily increase infiltration rates, although it can reduce sediment yields (Hogan and Grismer 2007). However, combined with surface tillage in the form of subsoiling or ripping, infiltration rates can be significantly increased. Mulch fills in the micro-basins left from the tillage operation (Figure 5.7).
Establish Vegetation — Ultimately, the best method to increase infiltration is to create conditions for a healthy vegetative cover. Good vegetative cover will produce soils with extensive root channels, aggregated soil particles, and good litter layers.
5.2.4 Road Drainage
Roads intercept surface and subsurface water and, depending on how the road is designed, either disperse or concentrate this water. Dispersed water is often seen on outslope roads, where water moves in sheets over the road surface during rainstorms. Concentrated water occurs where runoff from the road surface and cut slopes, as well as intercepted water from seeps and streams, is collected in ditchlines that flow into culverts or other road drainage structures. These structures, in turn, deliver the concentrated water to slopes below the fills. Concentrated water is not always directed into creeks or natural drainage landforms. Therefore, during rainfall events and snowmelt, concentrated water might be directed onto soils that were drier before construction. The greater water input to these sites increases soil moisture and may change the type of vegetation that can survive and grow.
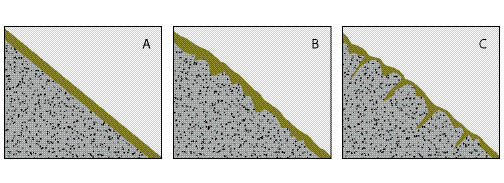
Figure 5.7 — Surface applied compost has greater surface area contact with the soil when it is applied to roughened surfaces (B), compared to smooth surfaces (A). Creating a rough surface prior to the application of composts creates better rooting, greater surface stability, and faster organic matter decomposition. Tilling the soil, through subsoiling and ripping, to depths of one to two feet (C) will break up compaction and create channels for compost to move into the soil, increasing soil contact and creating greater infiltration rates.
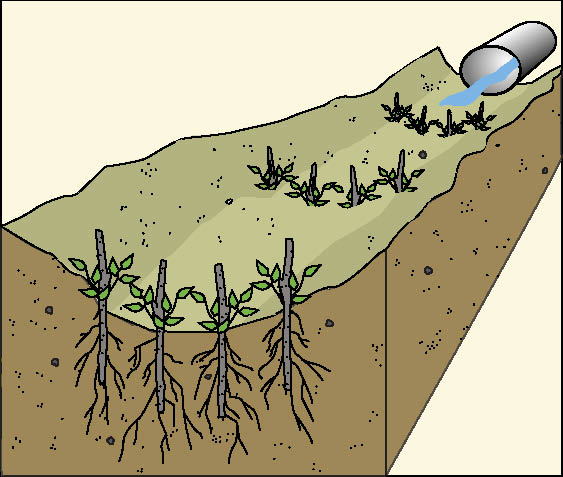
Figure 5.8 — In gullies, draws, intermittent streams, or below culvert outlets, live willow stakes (See Section 10.3.3.2, Live Stakes) are placed in rows, creating what is referred to as a live silt fence, to slow water velocities and catch sediment and debris (Polster 1997). The stakes root and establish into plants over time.
5.2.4.1 Road Drainage — How to Assess
Road drainage is assessed by identifying drainage structures on the road plans and visiting these sites in the field during both pre- and post-construction. The outlets of culverts are the areas most likely to have concentrated water that can be considered for mitigation. Outslope roads and long road shoulders will produce sheet water.
5.2.4.2 Mitigating for Road Drainage
Species Selection — In areas below culverts, soil moisture should be higher than surrounding areas after rainstorms or snow melt. These areas should be evaluated to determine if more moisture-loving plant species should be sown or planted to take advantage of the increased soil moisture. However, be aware that, in arid climates, these sites may be as dry as the surrounding areas during long periods of summer drought. Consider placing obstacles, such as berms or large wood, at the base of culverts and perpendicular to the slope to slow concentrated water and increase soil moisture in these areas.
Biotechnical Slope Protection — Gullies can form below culvert outlets, and, for this reason, these sites are often armored with rock. Moisture-loving vegetation, such as willows, sedges, and rushes, can be integrated into the hardened surfaces as shown in Figure 5.8 and as discussed in Section 10.3.3, Installing Cuttings.
Water Harvesting — Road surfaces, shoulders, and to a lesser extent, cut and fill slopes are impermeable surfaces that create runoff water during precipitation. Utilizing this water can be considered a form of water harvesting. Figure 5.4 shows a simple way of using water off road surface and shoulders.
5.3 Available Water Storage and Accessibility
The previous section discussed how water enters the soil surface. This section describes how water is stored in the soil, and how soil water is accessed by roots. The total available water-holding capacity (TAWHC) is the sum of all water stored in the soil profile that is available to plant roots. The amount of water that a soil can store is primarily a function of:
- Soil texture,
- Rock fragments,
- Soil structure,
- Rooting depth, and
- Mycorrhizae.
The amount of water a soil stores and how easily it is accessible by roots determines the types of species and the amount of vegetative cover a site can support.
5.3.1 Soil Texture
Soils are composed of minerals of varying sizes, ranging from clay (the smallest) to stone (the largest). Each mineral particle in a soil sample can be grouped into six categories depending on its size: clay (<0.00008 in [0.002 mm]), silt (0.00008-0.002 in [0.002 to .05 mm]), sand (0.002-0.08 in [0.05 to 2.0 mm]), gravel (0.08-3.0 in [2 mm to 8 cm]), cobble (3-8 in [8 to 20 cm]) and stone (>8in [20 cm]). The fine soil fraction is composed of a combination of sand, silt, and clay size particles. The proportion of these size groups in a soil is called the soil texture.
Figure 5.9 shows 12 soil textural classes by their proportions of sand, silt, and clay as defined by the U.S. Department of Agriculture classification system (Soil Survey Staff 1975). There are two other soil classification systems, American Association of State Highway and Transportation Officials (AASHTO) and the Unified Soil Classification systems, which are used for geotechnical engineering. These two systems use different particle size ranges and include parameters such as liquid limit and plasticity in classifying soils. There is no accurate way of converting values from these systems to the USDA textural classes.
Soil texture is an important function of soil water storage because the unique arrangement of pores created in each texture class holds differing quantities of moisture. Clays are typically thin, wafer-like particles with highly charged surface areas that retain large amounts of water. Clay particles are often arranged to form small void spaces, or micropores, that also store water. Sands, on the other hand, are large, rounded particles that have a very low surface area and therefore do not hold as much water. The large pores (macropores) that are created when sand particles are adjacent to each other are good for air and water flow, but poor for storing water. Soils high in silts hold more water than sands because of the greater quantity of micropores. However, silt particles are not charged, therefore holding less water than clays.
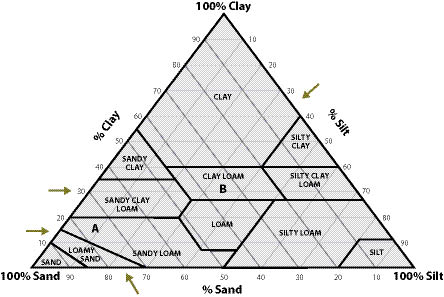
Figure 5.9 — The soil textural triangle defines 12 textural classes based on the percentage of sand, silt, and clay in a soil sample. The textural classes make it easy to describe soils without having to state percentage of sand, silt, and clay. To use the textural triangle, locate the percentage of sand on the bottom side of the triangle and trace the line up to the left hand side of the triangle. Do the same with either the silt or clay percentages on the other two sides of the triangle (follow silt diagonally down to the lower left and clay across from left to right). Where the two lines intersect is the textural class for that soil. For example, a soil with 75% sand and 15% clay would be a sandy loam (A). A soil with 30% clay and 35% silt would have a clay loam texture. Adapted from Colorado State University Extension Publication (GardenNotes #214 at http://www.ext.colostate.edu/mg/files/gardennotes/214-EstTexture.html).
5.3.1.1 Soil Texture — How to Assess
Soil texture can be determined fairly accurately in the field using the "feel" test. This is done with the aid of a soil sieve (2 mm opening size) and a bottle of water. Obtain a fairly dry field sample and separate the fine fraction from the coarse fragments with the sieve (note the volume of gravel in the sample). Take a sample of the fine fraction in the palm of the hand and moisten it with water. The soil is rubbed between the fingers and thumb and classified using the decision tree in Figure 5.10.
For a more exact determination of soil texture, a sample of soil can be sent to a soils laboratory for a particle size distribution test. This test will report the percentage of sands, silts, and clays in the sample. Published soil surveys of the project area will also give a reliable classification of the soil texture.
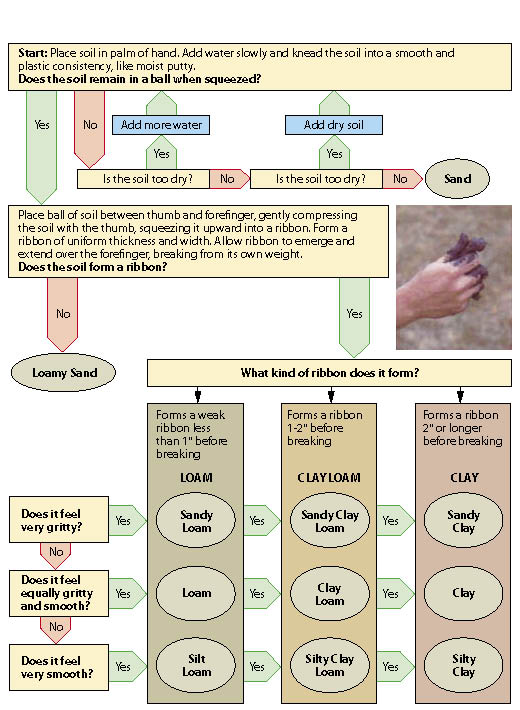
Figure 5.10 — Soil texture by feel method. Adapted from Colorado State University Extension Publication (GardenNotes #214 at http://www.ext.colostate.edu/mg/files/gardennotes/214-EstTexture.html).
Knowing soil texture is essential for estimating the available water-holding capacity (AWHC) of a soil. Figure 5.11 shows some typical available water-holding capacities for various soil textures. The values in this figure are generalized, but are acceptable for making recommendations on most revegetation projects. For a more accurate assessment, samples can be sent to soils labs for moisture determination. This is a specialized test and not all labs offer this test; therefore it is important to contact the lab prior to collecting samples. You can also measure water-holding capacity by the methods outlined in Inset 5.1.
5.3.1.2 Mitigating for Textures with Low Water-Holding Capacities
Organic Amendments — Incorporation of organic amendments (e.g., compost) can increase the water-holding capacity of a soil. Because the water-holding capacity of each type of organic matter varies by composition and degree of weathering, the effect on soil water-holding capacity by any organic matter being considered must be assessed prior to application (See Section 10.1.5, Organic Matter Amendments for assessment methods). Sandy textured soils benefit most from organic matter additions, especially those with plant available water of 9% or less (Claassen 2006), which are typically sands, loamy sands, and sandy loam soils. Testing several different rates of incorporated organic matter on soil moisture-holding capacity should be done to prior to selecting the source and the amount of material to apply.
Clay — The water-holding capacity of sandy textured soils can be increased by incorporating clay loam, sandy clay loam, and silty clay loam textures in the soil. The addition of clays should be at rates that result in new soil textures similar to loams, silt loams, or sandy clay loams. Higher rates of clay addition are not recommended. It is always important to test the additions of any soil to another to understand what the effects on water-holding capacity and structure might be. Ideally this should be in the field in small plots.
Polyacrylamides — Polyacrylamides are hydrophilic polymers that absorb many times their weight in water. They have been used to increase the water-holding capacity of greenhouse growing media. The use of polyacrylamides as a measure to improve impoverished soils associated with road construction, however, has not been demonstrated. The uncertainties of using polyacrylamides range from which of the many products on the market to use, the method of application, the life span under field conditions of each product, the effects of salts and minerals on water-holding capacity, and the amount of material to use. If the rate of polyacrylamide application in the soil is too high, the polyacrylamide granules will swell into the macropores, displacing soil air and creating anaerobic soil conditions (Cook and Nelson 1986). If the rate of application of polyacrylamides is too low, there is a good chance that there will not be much benefit to the soil. Fertilizers and minerals can reduce the amount of water being held (Wang and Greg 1990). Any full scale use of polyacrylamides should be tested at different rates on the site being revegetated. The best candidates for testing these products would be sandy textured soils.
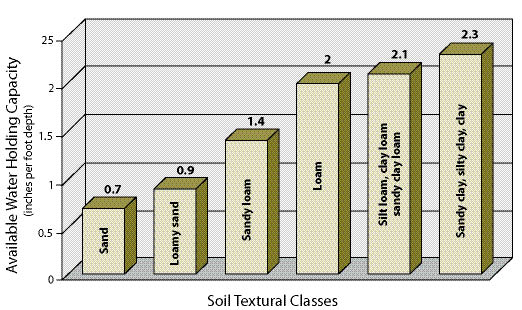
Figure 5.11 — General relationship between soil texture and available water-holding capacity. As clays increase in a soil, so does water-holding capacity. Typically, clay loam soils hold more than twice as much water as sandy textured soils (adapted after Ley and others 1994). The presence of humus in topsoil increases water-holding capacity of loams and sandy loams at a rate of 2.25% water to each percent rise in soil humus (Jenny 1980) which equates to approximately 0.75% increase in water for every 1% increase in organic matter.
5.3.2 Rock Fragments
Mountainous soils and highly disturbed sites are typically high in rock fragments. The presence of rock fragments is important because the rock reduces the amount of water and nutrients a soil can hold. At high volumes in the soil, rock fragments will limit the composition of species and vegetative cover a site can support.
The rock classification system classifies rock fragments into five size ranges: fine gravels (.08 to 0.2 in), medium gravels (0.2 to 0.8 in), coarse gravels (0.8 to 3 in), cobble (3 to 10 in) and stone (>10 in).
Highly weathered rock can retain some soil moisture depending on the size of the rock fragments and degree of weathering (Flint 1983). For practical purposes, however, it is usually assumed that the presence of cobbles and stone rock fragments in the soil will reduce the available water-holding capacity of the soil proportionally. For example, a sandy loam soil without rock fragments has a water-holding capacity of 1.4 inches per foot of soil (Figure 5.11). When 30% large rock fragments are added to the soil profile, the available water-holding capacity is reduced to 70% or 0.98 inches of available water (1.4 * 0.7 = 0.98). Fine and medium gravels (0.08 to .8 inches in diameter), on the other hand, hold some moisture. A rule of thumb is that these fine and medium gravels reduce water-holding capacity by two-thirds of their volume. In the above example, if 30% of the soil were composed of medium and fine gravels, the available water in this soil would be 1.12 inches per foot (1.4 - (1.4 * 0.3 * 0.66)).
5.3.2.1 Rock Fragments — How to Assess
Rock fragment content is usually determined in the field during soil surveys or soil sampling for laboratory analysis. Large rock fragments, such as cobble and stones, are estimated in a variety of ways. The most common methods are surveying freshly exposed road cuts or observing soil excavation during road construction. Estimating the volume can be difficult, and often the amount of rock is over- or under-estimated. One method of estimating large rock in road cuts is to take a digital picture and lay a grid over the surface, as shown in Figure 5.12. Whenever rock is estimated from old road cuts, it must be discerned whether a portion of the rock is masked by soil that might have moved over the rock. This is why it is best to observe freshly exposed road cuts.
Inset 5.1 — Measuring Available Water-Holding Capacity in the Field
(modified after Wilde and others 1979)
Available water-holding capacity (AWHC) can be measured in the field by collecting soil samples from a reference site or disturbed site in mid to late summer when soils are presumably as dry as they can be. For determining AWHC for undisturbed soils, samples are collected in bulk density rings in the same manner as sampling for bulk density (See Section 5.3.3). After removing the rings from the soil, cardboard or plastic is secured at each end of the rings to keep the soil from falling out. Rings are placed in airtight plastic bags to ensure that the samples stay intact during transport. The samples are weighed and placed in a bucket and filled with water to just the top of the ring. The sample is allowed to saturate with water. After you see glistening at the surface (indicating the soil is fully saturated), the ring is removed and placed upside down on paper towels and allowed to dry (soil surface should be in direct contact with towels). When paper towels become saturated with water, they are removed and replaced with dry towels. After 24 hours paper towels should not be saturated with water when in contact with the soil. The ring is then weighed. Available water-holding capacity (inches of available water per foot) = (wet weight — dry weight)/volume of cylinder * 12. For disturbed soils, the sample can be collected in an airtight bag and placed in a cylinder of known volume (placed firmly in the cylinder). The test is conducted from this point on as described above.
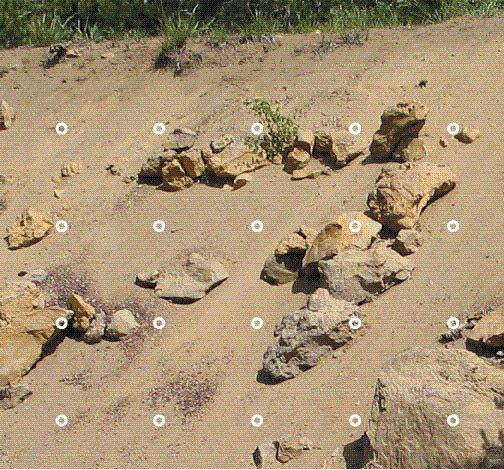
Figure 5.12 — The amount of rock in a section of soil can be roughly estimated from road cuts. Large rock can be determined by laying a grid of 20 circles over a photograph of a road cut and recording the number of circles intercepting rock (in the center of the circle). This value is divided by the total number of circles in the grid to obtain the percentage of subsoil in rock fragments. In the picture below, subsoil contains approximately 25% large rock (5 intercepted rocks divided by 20 points).
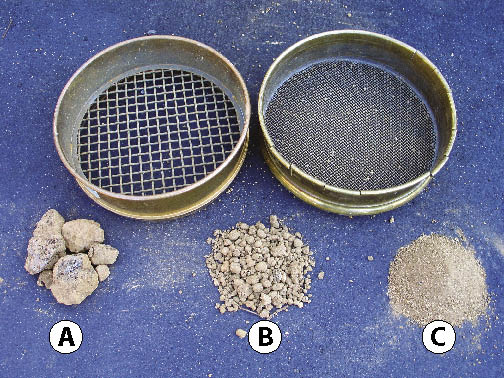
Figure 5.13 — The number 10 sieve (2mm opening) on the right separates soil particles (C) from rock particles (B and A). The 3/4 inch sieve on the left separates the fine and medium gravels (B) from the coarse gravels A).
Rock encountered while digging a soil pit will give a more accurate estimate of larger coarse fragments. Cobbles and stones, if they can be moved, are set apart from the soil when the pit is excavated. The volume of cobbles and stones is then visually compared to the volume of soil excavated from the soil pit to estimate the percentage of rock fragments.
Gravel content is determined from the excavated soil by sieving it through several soil sieves. Sieves are available through most engineering equipment companies (Figure 5.13). The 2 mm sieve (also referred to as a #10 sieve) is the most important sieve to use because it separates the gravels from the soil fraction. Another useful sieve is the 3/4 inch sieve because it separates the fine and medium gravels from the coarse gravels. This sieve can be used in the field to remove larger rock fragments from the soil sample to reduce the volume. Finer sieving can be done back at the laboratory with a 2 mm sieve. If the soils are dry, this can be done in the field. When soils are moist, they must be air dried first before they can be sieved. The gravel and soil fractions are weighed and the gravel weight is divided by the weight of gravel plus soil (multiplied by 100) to determine the percent gravel.
It is important to remember to include the volume of cobbles and stones estimated in the field with the gravels determined through sieving to calculate the total rock fragments in a soil:
% rock fragments in profile = (100 - % cobbles and stone) * % gravels in sample
For example, a soil is estimated to have 25% cobbles and stones from observing road cuts and from several soil pits. Sieving shows that 50% of the sieved soil is composed of gravels. The soil would be composed of 25% cobbles and stones, 37.5% gravels ((100 — 25) * .50), and 37% soil.
5.3.2.2 Mitigating for High Rock Content
Rock Removal — Removing rock fragments from the soil will increase the available water-holding capacity of a soil. This can be done through screening and reapplication of the screened material. Screened subsoil should be kept separate from topsoil in this operation. The greatest benefit from screening would be with soils that are very high in cobble and stone, where the reduction in volume of rock in the soil would be significant. The "grizzly feeder" acts as a giant sieve to sort rock from soil. Rocky soil is dropped on the surface of the grizzly and fine soil falls through the screen while the rock fragments roll to the side through gravity or vibratory action, depending on the type of grizzly.
Incorporate Compost — Compost incorporated in the soil at high rates will increase the water-holding capacity of a rocky soil (See Section 10.1.5, Organic Matter Amendments). Depending on the size of the coarse fragments, incorporation can be difficult.
Surface Apply Compost — A more practical method to mitigate for rocky soils is to apply composts to the soil surface without mixing. When surface applied, composts can be good growing media for seeds of grasses and forbs (See Section 10.1.3, Mulches). At rates of greater than 3 inches applied to the surface, seeds can germinate well and establish into seedlings that can access moisture and nutrients not only from the compost, but also some moisture from the rocky soil below the compost.
Apply Topsoil — If topsoil is available, it can also be applied over a rocky soil (See Section 10.1.4). Topsoil depth will have to be placed deep enough to compensate for the quantity of rock in the soil being covered.
Planting Islands — On very rocky sites, mitigation of rocky soils can be accomplished by focusing mitigating measures into planting islands (See Section 10.1.8.4). Topsoil application, compost additions, or rock removal can occur in mounds, pockets, or benches strategically located throughout a revegetation unit and then planted with trees and shrubs.
5.3.3 Soil Structure
Just as soils are composed of many-sized mineral particle sizes, they are also composed of different size voids, or pores, whose influence is responsible for water movement, water storage, air flow, and root penetration. Small pores (micropores) strongly influence soil moisture-holding capacity, while large pores (macropores) are responsible for water movement, air flow, and root penetration. The arrangement of large pores is called soil structure. It is qualitatively observed as cracks, channels, aggregates, crumbs, and clods in the soil, and described by alternative terms such as friability and tilth. Water flow and root penetration depend on good soil structure. If soil structure is poor or compacted, roots are less able to penetrate the soil to access the water. Soil structure is important for other soil functions such as air flow, drainage, permeability, infiltration, and as essential habitat for soil life. Soils with good structure are typically very productive.
Soil structure is significantly reduced by heavy equipment on soils. The pressure applied by the equipment compacts the macropores (large pores), reducing soil volume and increasing soil density. This impact is called soil compaction (Figure 5.14). The effects of soil compaction on tree growth are well documented (Poff 1996). Trees growing on highly compacted soils have far less root, stem, and leaf production than those growing on non-compacted sites. Studies have shown a linear relationship between the increase in surface soil bulk density and decrease in height growth of young Douglas-fir and ponderosa pine trees (Froehlich and McNabb 1984). It should be assumed that soils will be highly compacted after construction due to the use of heavy equipment. In addition to reducing the potential of a construction site to grow vegetation, compaction increases runoff and sedimentation in rainstorm events. During the growing season, this means there will be less water entering the soil after rainstorms.
Compaction can occur several feet below the soil surface, depending on the type and weight of the equipment, soil texture, and soil moisture content. Very compacted strata can significantly reduce or eliminate root penetration. Where compacted strata occur, downward water movement is restricted and water may saturate the soil layers above the compacted layer. The resulting saturated soil conditions can be very restrictive to root growth because of the lack of oxygen and the propensity for higher incidence of disease (Steinfeld and Landis 1990) and ultimate mortality (Figure 5.15). Compacted layers will naturally recover to their original porosity through root penetration, animal activity, and freeze-thaw events, but recovery can take 20 to 70 years (Wert and Thomas 1981; Froehlich and others 1983).
5.3.3.1 Soil Structure — How to Assess
It is easy to differentiate good soil structure from compacted soil (Figure 5.14) by touch or sight, but measuring it quantitatively is difficult. There are several indirect field tests to quantify soil structure. These include bulk density and penetrometer tests. It is important to consider that exact soil measurements are less important than recognizing that after construction, most soils will be compacted. Nevertheless, these tests are good to perform when there is a question of degree of compaction, during reference site assessment, or when assessing the effects of revegetation treatments over time.
The bulk density test measures the dry weight of a standard volume of soil. If the soil has a high porosity, the bulk density values will be low; if the soil is compacted, the bulk density will be high. In this method, a cylindrical tube is driven into the soil with a portable bulk density sampler and a soil core is removed. The soil is shaved evenly on both ends so that the soil is exactly the shape and volume of the cylinder. The soil is then removed from the cylinder, oven-dried and weighed.
Bulk Density = weight of soil (g) / cylinder volume (cc)
Bulk density values of a disturbed site must be related back to the bulk density of an adjacent reference site to make the values meaningful. Remaining within a 15% increase in bulk density over reference site values is ideal. Unfortunately, the bulk density method is time consuming and cannot be conducted on soils with high rock fragments.
A less quantitative, but more practical, method of measuring soil porosity is with a soil penetrometer. This equipment measures soil strength instead of density. Compacted soils have greater strength, and greater resistance to penetration by a penetrometer, than non-compacted soils. There are several types of penetrometers that can be purchased for field work-penetrometers that measure the resistance as a continuous pressure is applied to the probe and penetrometers (impact penetrometers) that measure the number of blows of a hammer to drive the penetrometer into a specified depth. A monitoring protocol for assessing compaction using an impact penetrometer has been developed by the NRCS (Herrick and others 2005b). The most practical and economical field method for assessing compaction, however, is simply using a long shovel as shown in Figure 5.17. In this method, a site is traversed and, at predetermined intervals, a shovel is pushed into the ground to determine how loose the soil is. By applying the entire body weight to the shovel and observing the distance the shovel penetrates the soil, a qualitative measurement of soil compaction can be made. A rule of thumb is that a shovel penetrating over 12 inches deep indicates that a soil has a very high porosity; penetration below 3 inches deep indicates a very low porosity.
Whether a shovel is used or a soil penetrometer, the readings are affected by rock content and soil dryness. When soils are dry, they have more strength and higher resistance to penetration. This is why any comparative sampling using a penetrometer must be done at the same moisture levels. Encountering rock poses an obvious problem to the use of penetrometers. It can be overcome by moving the penetrometer around until a point is found where the penetrometer can be applied without hitting rock.
Penetrometer values for disturbed sites must be evaluated against those from reference sites for comparative analysis.
5.3.3.2 Mitigating for Poor Soil Structure
Tillage — Breaking up compacted layers can be done effectively when deep tillage equipment is operated correctly (See Section 10.1.2, Tillage).
Incorporate Organic Matter — The effectiveness of deep tillage can be enhanced if organic matter is incorporated into the soil prior to tillage (See Section 10.1.5, Organic Matter Amendments). Organic matter can keep the soil from settling back to higher, pre-tillage densities. Application rates at which organic matter showed positive effects on soil structure was observed at a ratio of 25% incorporated organic matter to 75% soil by volume (Claassen 2006). Longer shreds of organic matter are preferred over smaller, chip sizes because the longer strands create interconnecting pathways for water, air, and roots while increasing soil strength (Claassen 2006). The additions of non-composted organic matter, however, will tie-up nitrogen for a period of time. While this might be problematic in the short term, the importance of developing soil structure for long-term site recovery might override the concerns about the lack of immediately available nitrogen.
Operate Equipment With Care — Soil compaction is greatest when soils are moist. When heavy equipment is being used on non-compacted soils, the moisture status of the soil must be considered. Under moist and wet conditions, soil structure may be severely damaged. If heavy equipment must be used, schedule it during times when the soil is dry. Compaction can be also be minimized by using smaller equipment (Amaranthus and Steinfeld 1997). Leaving slash or deep mulch on the soil surface can further reduce the amount of compaction because this material will provide some cushioning to the soil.
Avoid Last Minute Compaction — There is no avoiding soil compaction during construction, but compacting the soil after mitigating treatments have been implemented must be avoided. There are many cases where the benefits of mitigating treatments have been nullified by the lack of attention to heavy equipment operations after topsoil additions or tillage treatments have been made. For example, topsoil salvage and placement, as discussed repeatedly in this manual, benefit the site in many ways. But this expensive mitigating measure loses much of its value if the soils are compacted during or after soil placement. Once topsoil is deep-tilled, there must be no more passes by equipment over the soil.
5.3.4 Rooting Depth
Rooting depth is the distance from the surface of the soil to the lower reaches that roots can penetrate. It encompasses any strata that can be accessed by plant roots (topsoil, subsoil, and parent material). The deeper the rooting depth of a disturbed site, the greater the total available water storage and the higher the productivity of the site.
Rooting depth is affected by restrictive layers that block root penetration to lower strata (See Section 5.7.2, Restrictive Layer). For example, the rooting depth of a post construction site is estimated at 6 feet deep. However, further investigation finds that there is a highly compacted layer at 12 inches, which would limit most, if not all, root penetration below that point. The rooting depth under these conditions has been reduced to only one foot of soil instead of six feet. Restrictive layers also include soils with very high or low pH, toxic materials, or a high water table.
Rooting depths vary by plant species and age of the vegetation. Most mature tree species have deep root systems that access subsoil and parent material; roots of grasses and forbs are predominantly limited to the surface soils. Annual grasses and forbs require less rooting depth than perennial grasses and forbs, with the roots of these species growing in the upper surfaces of the soil. The age of the vegetation also determines the abundance and location of roots. Newly established seedlings have shallow roots but, as the plants mature, root systems expand to access moisture deeper in the soil.
Rooting patterns and root morphology play a role in how plants access soil water. Some species have finer textured root systems that access tightly held soil moisture; other species have aggressive root systems that can penetrate deeply into cracks between rock fragments. Grasses, for instance, have shallower root systems than trees and shrubs, but their small size and high density in the surface soil gives them an advantage in shallow soils.
5.3.4.1 Rooting Depth — How to Assess
Rooting depth should be estimated from reference sites during planning and post-construction, but is not always an easy parameter to measure. Observing road cuts is often the best means to determine rooting depth below the topsoil and subsoil. Rock type (e.g., granite, sandstone, schist), fracturing patterns, rock weathering, and the degree of rock fracturing will give an indication of rooting depth. Observing the amount and type of roots in the fractures of existing road cuts will give a good idea of rooting depth.
Fracturing and weathering of rock can also be determined from geotechnical analysis. If the bedrock has been drilled, the drill log report can give an indication of degree and depth of rock fracturing and weathering. One way that rock quality is assessed is through a classification called the Rock Quality Designation Index (RQD). This system rates the bedrock by how much fracturing is observed in the cores. It is calculated by measuring the pieces of rock in the core sample that are longer than 10 cm, summing the length of these pieces, and dividing by the total length of the core (Deere and Deere 1988). A small RQD means that the bedrock is highly fractured whereas a high RQD means the bedrock is massive. A RQD may be poor from an engineering standpoint because of the high fractures, but favorable from a revegetation standpoint because cracks will hold some moisture and allow root penetration. A RQD rated as "very poor," "poor," and even "fair" should be somewhat favorable to root penetration.
Rooting depth is also affected by the presence of a restrictive layer caused either naturally or by compaction. How to determine the presence of these layers is addressed in Section 5.3.3.1 and Section 5.7.2.1.
There are many references in the literature defining the depth of soil needed to support different plant communities. For example, 18 inches of soil has been shown to support simple grassland ecosystems, but more diverse native grassland communities are reported to require up to 4 feet or more (Munshower 1994). These figures can be misleading if they are not put in the context of site climate and soil type. In many respects, it is more useful to state the total available water-holding capacity (TAWHC) of a site rather than the rooting depth.
Figure 5.18 shows how the TAWHC is calculated. Using the same format and equations, a similar spreadsheet can be created for your use by copying the equations into the "Results" column. In this example, there is 1 foot of topsoil and 2 feet of subsoil over a highly fractured basalt. The topsoil has a loam texture and available water-holding capacity of 2.0 inches (estimated from Figure 5.11 or obtained from lab results) but, because of the rock fragments, it is reduced by approximately 0.4 inches. The subsoil has a high water-holding capacity because of high clay content, but the available water-holding capacity is reduced by half due to rock. Highly fractured basalt is encountered at a depth of 2 feet, and it is estimated from the road cut that approximately 30% is actually fractured. Within these weathered fractures is a gravelly clay loam textured material storing approximately 0.5 inches of water. The TAWHC for this site would be the sum of all sections of soil (approximately 3.2 inches).
The TAWHC can be used for relative comparisons between revegetation units and reference sites. For example, the TAWHC for a post construction soil is 3.6 inches compared to an adjacent reference site, which is 6 inches. If the desired future condition of the post construction soil is to be similar to the adjacent reference site, then the TAWHC of 3.6 inches must be increased upward toward 6 inches for the site to be capable of supporting the vegetative community occurring on the reference site.
5.3.4.2 Mitigating for Poor Rooting Depth
Increase Available Water-Holding Capacity — Improving the water-holding capacity of the existing soil will increase TAWHC. Mitigating measures discussed in Section 5.3.1, Soil Texture and Section 5.3.2, Rock Fragments can be used to increase soil moisture.
Tillage — If restrictive layers due to compaction are encountered, deep tillage should be considered. Section 10.1.2 provides guidelines for deep tillage.
Apply Topsoil — Increasing rooting depth and TAWHC can be accomplished by applying topsoil (See Section 10.1.4).
Planting Islands — Mitigating measures, such as applying topsoil, organic matter incorporation, deep tillage, and other measures that increase water-holding capacity, can be focused in strategic locations, such as planting islands. This will conserve on materials and reduce costs (See Section 10.1.8.4).
Blasting — Strategic blasting to shatter the parent material has been suggested (Claassen 2006) as a possible means of increasing rooting depth.
5.3.5 Mycorrhizal Fungi
The discussion to this point has addressed the primary factors responsible for soil water storage (soil texture, rock, and rooting depth) and the accessibility of roots to grow to this water (soil structure). In this section, the discussion turns to how plants increase the efficiency of accessing water through mycorrhizae. While mycorrhizae provide many other benefits to the site besides water enhancement, they are covered in depth in this section because of the importance of water to establishing vegetation on highly disturbed sites in the western United States.
Mycorrhiza is the unique symbiotic relationship between fungi (called mycorrhizal fungi) and host plants. To the naked eye, many mycorrhizal fungi appear as a fine web or netting that seems to connect the root system to the surrounding soil (Figure 5.19), and in essence, this is exactly what is occurring. The extremely small hyphae of the mycorrhizal fungi are actually taking on the form and function of an extended root system of the plant. Because mycorrhizal hyphae are up to five times smaller than plant roots, they are able to access spaces in the soil not easily accessible by the larger plant roots. Mycorrhizal hyphae not only provide the plant with a greater access to soil moisture and nutrients, they also surround and protect roots from soil pathogens. In return, the host plant supplies carbohydrates to keep the mycorrhizal fungi alive.
Mycorrhizae play a critical role in site restoration by building soil structure. Hyphae and water stable organic "glues," like glomalin, are excreted by the mycorrhizal fungi and bind soil particles together into aggregates. These aggregates stabilize the soil, improving soil structure (See Section 5.3.3, Soil Structure) important for good air exchange and water permeability. This basic soil building process, or repair, facilitates the creation of nutrient reserves and nutrient cycling essential for restoring ecosystems (Miller and Jastrow 1992). Mycorrhizal fungi can also improve survival of tree and grass seedlings (Steinfeld and others 2003; Amaranthus and Steinfeld 2005). A healthy population of mycorrhizal fungi has also been shown to increase plant biomass and cover (Wilson and others 1991; Brejda and others 1993; Sobek and others 2000), and increase the diversity of native species (Smith and others 1998; Charvat and others 2000).
Ninety percent of all terrestrial plants form symbiotic relationships with mycorrhizal fungi. Of the thousands of known species, most generally fall into two categories — ectomycorrhizal fungi and arbuscular mycorrhiza fungi.
Arbuscular mycorrhizal fungi (AMF), formerly called endomycorrhizae, are the most commonly occurring mycorrhizal fungi, forming on 75% to 85% of plant species. These include legumes, composites, grasses, bulbs, most shrubs, and ferns. In addition, AMF occur on many tree species, including redwoods and some cedars, and many types of tropical trees. AMF grow inside the roots of the host plant and extend hyphae out into the soil. These fungi are more general in their association with plant species, meaning that one mycorrhizal species can form an association with a broad spectrum of plant species. AMF reproduce in two ways: 1) by forming single spores outside of the root, and 2) from fungal structures (vesicles and hyphae) present inside a colonized root system. Arbuscular mycorrhizal fungi do not disperse their spores in the wind, but instead are dispersed from root to root or by animals. For this reason, recolonization of drastically disturbed sites by arbuscular mycorrhizal fungi can be slow, especially if there are limited sources of healthy, undisturbed soils nearby to repopulate the site.
Unlike arbuscular mycorrhiza fungi, ectomycorrhizal fungi, as the name implies, coat the outside of the roots with hyphae that extend out into the soil. Ectomycorrhizal fungi form on 5% to 10% of plant species, the majority of which are forest trees in the western United States. Species include Douglas-fir, western larch, true firs, spruce, hemlock, oak, manzanita, willows, and cottonwood. These fungi form a netting of fine hyphae around the root system of the tree that is often observable on nursery produced seedlings inoculated with mycorrhizal spores. Unlike AMF, the relationship between ectomycorrhizal fungi and host species is very specific. Many ectomycorrhizal fungi species have evolved to associate with only one plant species. Ectomycorrhizal fungi produce fruiting bodies, such as mushrooms, puffballs, and truffles, that yield reproductive spores for wind or animal dispersal.
AMF and ectomycorrhizae do not associate with all plant species found in the western United States. Manzanita and madrone, for instance, form arbutoid mycorrhizae, while huckleberry form ericoid mycorrhizae. The remaining 10 % of plant species do not form mycorrhizae at all. Many of these plant species have evolved root systems that function similarly to mycorrhizae and therefore can outcompete mycorrhizal species during early establishment on highly disturbed sites. While host plants might be present during early establishment of these sites, they nevertheless languish because of the lack of mycorrhizal fungi, giving non-mycorrhizal species a distinct advantage. This advantage is why many plant species that are considered weeds are non-mycorrhizal species.
5.3.5.1 Mycorrhizal Fungi — How to Assess
Where soils have been drastically disturbed, it can be assumed that the mycorrhizal fungal propagules that colonize plant roots are drastically reduced or absent from the site. The size and severity of the disturbance determines the colonization rates of mycorrhizal fungi. As the level of disturbance increases, the density of viable fungi propagules typically decreases. Small disturbances surrounded by native forests or rangelands often reestablish quickly; in larger disturbances, where topsoil has been removed, recolonization by mycorrhizal fungi may take years.
Some laboratories offer testing for mycorrhiza fungi, but these are expensive tests. Since it is unlikely that mycorrhizal fungi will be found in recently disturbed sites lacking topsoil, conducting these tests for most projects are unnecessary.
5.3.5.2 Mitigating for Lack of Mycorrhizal Fungi
For most construction projects, the management of mycorrhizae should be considered in the early stages of project planning. Several strategies are available to enhance mycorrhizal colonization.
Minimize Soil Disturbance — Operations that maintain topsoil will often preserve mycorrhizal inoculum and maintain soil nutrition. Partially disturbed topsoil is often adequate for reestablishing mycorrhizal plant species. Partial disturbances include clearing and grubbing of road right-of-way vegetation, ground-based logging, and light to moderate intensity burns. Colonized root systems left behind in these operations are sources of inoculum for endomycorrhizae.
Leave Undisturbed Areas — The colonization of AMF into highly disturbed sites is slow. Spores are transported by soil erosion and animal movement, but not by air. By leaving small areas of native vegetation and undisturbed soils within the larger disturbance, travel distance for the spread of fungi is reduced, facilitating a quicker repopulation of AMF. This practice is especially important where the size of the disturbance is large.
Salvage Topsoil — Reapplying topsoil to drastically disturbed sites is commonly done when quality native topsoil is available (See Section 10.1.4, Topsoil). If topsoil is obtained from non-forested sites, such as meadows, rangelands, and unforested clearcuts, AMF is often abundant because the host plants are grasses and forbs. It should not be assumed, however, that there will be ectomycorrhizal spores present to colonize the roots of tree seedlings.
Apply Topsoil to Planting Holes — If topsoil is very limiting, placing healthy topsoil into holes prior to planting seedlings is an effective method of introducing an inoculum to a disturbed site. Collecting soils as inoculum from young, actively growing forests has been shown to be suitable inoculum for young tree seedlings (Amaranthus and Perry 1987).
Apply Commercially Available Mycorrhizal Fungi Inoculums — Applying commercially available mycorrhizal fungi inoculum is another method used to repopulate highly disturbed sites (See Section 10.1.7, Beneficial Soil Microorganisms). Commercially available sources of mycorrhizal inoculums are available for ectomycorrhizal and AMF plant species. These inoculums can be applied in hydroseeding slurries, as seed coats and root dips, through irrigation systems, or incorporated into the soil by broadcasting or banding. When purchasing live plants from a nursery, rooting media can be inoculated with mycorrhizal fungi during nursery culture (Figure 5.20). Fine grades of mycorrhizal inoculum can be applied to the surface of the soil and will move into the soil surface with rainfall. Coarser textured commercial inoculums must be incorporated in the soil to make them effective.
Reduce Fertilizer Use — While the application of fertilizer can increase plant biomass in the short term, it can also suppress mycorrhizal infection (Jaspers and others 1979; Claassen and Zasoski 1994). However, low rates of fertilizer have been shown to help establish plant cover and improve mycorrhizal colonization (Claassen and Zasoski 1994).
5.4 Water Loss
Water loss is the depletion of soil moisture during the year through transpiration (loss of water through leaves/needles) and evaporation of soil moisture from the soil surface. The rate at which evaporation and transpiration draw moisture from the soil profile is the evapotranspiration (ET) rate. ET rates can change daily, weekly, and seasonally: 1) daily changes occur as the sun hits vegetation, temperatures and wind speeds rise, and ET rates increase; 2) weekly changes occur when ET rates rise and fall as weather systems change from hot and dry to cool and wet; and 3) seasonal changes occur as air temperatures rise in spring, reaching maximum temperatures and minimum humidities in the middle of the summer, then decline in the fall (Figure 5.21).
Water loss due to ET can be influenced by a number of abiotic and biotic factors, primarily:
- Wind,
- Site aspect,
- Competing vegetation, and
- Soil cover
On both disturbed and undisturbed sites, the effects of wind patterns and aspect on ET rates influence the types of species that can survive and grow. Competing vegetation that colonize a site following disturbances can have a large influence on the amount of soil moisture stored and the availability to desirable plants.
Evaporation of moisture from the soil surface is not generally a major factor on undisturbed soils because they are often protected by a surface mulch of litter and duff. These soil covers allow rainfall or snowmelt into the soil, but create a barrier to surface evaporation. Surface evaporation becomes a factor when these protective covers are removed or destroyed during construction.
Consider the plant as the "middleperson" between the atmosphere, which demands water from the plant, and the soil, which acts as a bank of moisture from which the plant is able to draw. When the atmosphere is demanding very little water (low ET rate), the plant easily pulls water from the soil to the leaves. Over time, as soil moisture becomes depleted, the plant comes under greater stress. Not only is the atmosphere demanding greater moisture from the plant, but less moisture is available. Consequently, the plant comes under very high moisture stress. The amount of stress that a plant is under is referred to as plant moisture stress or PMS (Figure 5.23). PMS is at its highest from middle through late summer in the western United States, when the ET rates are at their highest and soil moisture levels at their lowest.
5.4.1 Wind
Wind is often overlooked as a factor in the success or failure of reestablishing native vegetation, but it can play a major role, especially on sites where summers are hot and dry and soil moisture levels are low. Until seedlings become established, high winds can severely limit growth and can ultimately lead to mortality.
5.4.1.1 Wind — How to Assess
Wind speed equipment is available, but most likely too costly for most revegetation specialists. Site visits during different times of the year can give some indication whether wind is a problem. Other site characteristics, such as position on the slope (e.g., ridgelines are more prone than valley floor), or proximity to forested environments — as forests often reduce wind speeds, — can be used to infer wind strengths and directions. In many environments, prevailing winds often come from one, or sometimes two, directions. Existing vegetation can sometimes give clues to prevailing wind directions (e.g., trees bent away from the prevailing wind). County and state department of transportation employees are often resources for local weather information. Recognize that road construction itself may change wind patterns (e.g., creating a wind tunnel by constructing a throughcut or removing a swath of existing vegetation). Some visual indicators of wind erosion are wind scour (See Figure 5.40 in Section 5.6), exposed roots, and deposition areas.
5.4.1.2 Mitigating for High Wind
Road Design — Designing islands of undisturbed vegetation to help break up wind patterns can aid vegetation establishment. The taller the plants left undisturbed, the greater the wind protection. Established trees, particularly those with low-growing branches, give the greatest protection from wind.
Wind Barriers — Obstacles that block wind at the soil surface can be effective for early seedling survival. These obstacles can include stabilized logs, large rocks, berms, and stumps. In using these structures, seedlings should be planted on the windward side.
Tree Shelters — Tree shelters completely surround seedlings and block them from the wind (See Section 10.4.4, Tree Shelters). They are an effective means of reducing ET rates created by high winds during early establishment. Once the vegetation has emerged from the top of the tube, however, tree shelters no longer protect the emerging foliage from the wind.
Shade Cards — Shade cards are sometimes used to block wind, but they are less effective than the fully enclosed tree shelter (See Section 10.4.3, Shade Cards). When used to block wind, shade cards must be placed on the windward side of the seedling, which is not necessarily the same location that cards would be placed if protection from sun is the objective. Often two shade cards are placed around the seedling for added protection against the wind. Placement of the shade cards at the height of the foliage affords greater protection to the seedling.
Appropriate Species Selection — The drying and damaging effects of wind are important considerations in appropriate species selection. A simple assessment of soil type and rainfall may not account for the effects of wind. Choosing hardier, wind-tolerant, and more drought-tolerant species may be necessary to establish vegetation on windswept sites. Find reference sites in windy locations to indicate which species are adapted to wind.
Leave Surface Roughened — A roughened soil surface can create a micro-basin or relief that protects young germinants from the drying effects of wind during the establishment phase (See Section 10.1.2, Tillage).
5.4.2 Aspect
Aspect is the direction a slope is facing, and is one of the predominant site characteristics affecting evapotranspiration. South and west aspects receive more solar radiation during the day and have higher ET rates than north and east slopes, and are therefore warmer and drier. Soils on these sites dry out faster than north and east slopes. In spring, during seed germination, south and west aspects can dry very quickly between rainstorms, reducing the rates of germinating seeds. As seedlings emerge and grow through spring and early summer, temperatures on the south and west slopes continue to rise to very high levels, creating very unfavorable conditions for seedling establishment. Even with planted seedlings, high surface temperatures can damage stems near the ground line, severely affecting seedling survival and establishment (Helgerson and others 1992).
In moist, cool climates, where moisture is not the limiting factor, south and west slopes are often very productive and have greater cover. Warmer soil and air temperatures create a longer growing season, offsetting the effects of moisture stress on plant growth.
On high elevation sites, north and east aspects are cold compared to the south and west aspects. The growing season is much shorter, resulting in very different composition of species between aspects. At high elevations, soil temperatures on south and west slopes stay higher longer in the fall, providing the opportunity to plant in the late summer and early fall months (Figure 5.24). During the spring at all elevations, south and west slopes warm up much sooner than north slopes. The differences in soil temperatures between north and south aspects is a consideration for determining when to plant.
5.4.2.1 Aspect — How to Assess
Aspect is measured in the field by facing the fall line of the slope (the imaginary line a ball would roll) and taking a compass bearing downslope. A "northeast slope," "northeast aspect," "northeast exposure," or "northeast-facing slope" all refer to an aspect with a compass bearing facing northeast.
Aspect can also be measured from topographic maps by drawing an arrow perpendicular to the contour lines and pointing the tip of the arrow downslope. Aspect is often a factor for delineating one revegetation unit from another due to the strong influence on growth and survival of seeds, seedlings, and cuttings. It can be useful to differentiate revegetation units using aspect by highlighting all areas on the road plan map with south to west slopes.
Soil and air temperatures differ greatly between aspects; taking temperature measurements can be important for assessing the effects of aspect on revegetation. There are many types of recording devices available on the market, but only equipment that can download data to spreadsheets for analysis and graphing should be considered. Some equipment has become so inexpensive that more than one unit can be purchased (Figure 5.25).
5.4.2.2 Mitigating for South and West Aspects
For most sites, any treatment that will shade vegetation on the south and west slopes from intense solar radiation should increase survival and growth of establishing plants.
Overstory Vegetation — Keeping overstory trees at a minimum density of one tree per tenth acre is a rule of thumb for reducing soil temperatures below lethal levels on south aspects (Helgerson and others 1992).
Shade Cards — Shade cards can significantly increase seedling survival on south aspects (Hobbs 1982; Flint and Childs 1984) (See Section 10.4.3, Shade Cards). They must be placed close to planted seedlings so that the stem and lower portion of the seedlings are shaded from the afternoon sun (Helgerson and others 1992).
Obstacles — Large obstacles that cast significant amounts of shade on young seedlings will create a more favorable environment for seedling establishment and increase seedling survival (Minore 1971). These include stabilized logs, large rocks, berms, and stumps. Seedlings should be planted on the north and east side of these features to be shaded from the afternoon sun.
Figure 5.24 — Site climate changes throughout the year depending on aspect. | ![]() |
![]() |
Figure 5.25 — Temperature recording technology has become smaller and very inexpensive. The iButton® shown next to the nickel can record more than a year of temperature data. |
![]() |
Mulch — On south exposures, the use of mulches as a moisture barrier should be considered for seedlings, seeds, and cuttings (See Section 10.1.3, Mulches).
Species Mix — The composition of species will probably be different for north and south aspects. Species adapted to hotter and drier environments are used for revegetating south exposures; those adapted to cool, moist environments are used on north aspects. Elevation can offset the effects of aspect. For example, species that grow on low elevation, north aspects often occur several thousand feet higher on south aspects because of the difference in temperatures. Reference site vegetation surveys will guide the revegetation specialists in the selection of appropriate species for each exposure.
Plant Material Rates — South aspects often require a higher density of seedlings, cuttings, and seeds than north aspects due to the expected higher mortality rates. Adjusting for increased mortality rates is made when calculating plant materials rate for seeds (See Section 10.3.1), cuttings (See Section 10.2.2.5), and plants (See Section 10.2.5).
Planting and Sowing Windows — Take advantage of warmer spring and fall soil temperatures on the south exposures by sowing and planting earlier on these sites (See Chapter 6).
5.4.3 Competing Vegetation
Controlling competing vegetation, whether native or non-native, around planted seedlings reduces the rate at which water is withdrawn from the root zone and increases the potential for survival and growth. A major factor affecting seedling survival and growth is the timing and the extent to which roots of competing vegetation encroach into the soil around the roots of the planted seedling. Annual grasses and forbs generally germinate early in the season as soil temperatures warm, often before native species. The early establishment of annual grasses and forbs deplete surface soil moisture, making it harder for perennial species to become established. Cheatgrass is an example of an annual species that establishes quickly in the early spring and depletes the soil moisture in the spring as perennial species are just beginning to germinate.
The rate at which water is depleted is a function of the type of competing species. Grass species, for instance, have very fibrous root systems in the surface soils which allow them to withdraw moisture very quickly and efficiently during dry weather. Unless grasses are controlled, it is very difficult to achieve good survival of planted seedlings in areas with high densities of grass. Perennial forbs are generally less competitive than grasses because their root systems are deeper and less concentrated in the surface where the seedlings are withdrawing moisture.
5.4.3.1 Competing Vegetation — How to Assess
Predicting the potential for vegetative competition at the time of planting on highly disturbed sites is difficult at best. If disturbed sites have been included as reference areas, some predictions of competing vegetation can be made. If competing vegetation becomes established, methods described in Chapter 12 for measuring ground cover can be used for assessment.
5.4.3.2 Mitigating for Competing Vegetation
Do Not Wait to Revegetate — Competing vegetation increases over time. The first year after construction is the optimum period to establish vegetation from seeds, seedlings, or cuttings. By the second or third years, competing vegetation is likely to dominate the site if no revegetation treatments have been applied. If competing vegetation dominates the site before native plants have become established, the measures to control the competing vegetation become far more expensive and less effective.
Scalping — Removal of competing vegetation by manually scraping or scalping the soil surface to remove weeds is ineffective in increasing seedling survival on dry sites when the area scalped is small (<2 foot radius); scalping becomes effective when the clearing is very large (2 foot radius or greater). Some of the problems associated with scalping are: 1) topsoil is removed; 2) surface soil evaporation is high; 3) weeds can invade on the bare soil; 4) infiltration rates are low; and 5) soils are prone to erosion. Scalping becomes more effective when the treated soils are immediately covered with a mulch or weed barrier cloth.
Mulches — Mulches have been shown to increase survival and growth of newly planted seedlings by reducing the amount of moisture loss through evaporation and plant competition (DeByle 1969; Lowenstein and Ptikin 1970; Davies 1988a, 1988b; Helgerson and others 1992). There is an array of mulches that can be used around seedlings (See Section 10.1.3, Mulches).
Herbicides — The use of herbicides for controlling competing vegetation around seedlings has been shown to be effective in increasing survival (Childs and Flint 1987). Not only is soil moisture increased in the rooting area of the planted seedling due to reduced transpiration, but dead foliage acts as a mulch or shade, further protecting the soil from moisture loss. There is a risk in using herbicides around shrub or tree seedlings because most herbicides are toxic to these species. The result of using herbicides may range from lower growth rates in subsequent years to increased mortality in the desirable species. This risk can be reduced by 1) using soil-active herbicides, 2) using extreme care when applying herbicides, 3) utilizing herbicides low in toxicity to the planted species, and 4) applying them during periods when the seedlings are less susceptible to damage (Helgerson and others 1992). If the road is on public lands, the land managing agencies should be contacted to discuss policies regarding the use of herbicides. Herbicides must only be applied by a trained professional following manufacturer and government guidelines and regulations for safe application and use.
5.4.4 Soil Cover
The thickness and composition of material that covers the soil surface influences many important soil properties covered in this manual, such as infiltration rates, interception losses, soil temperatures, surface erosion, runoff, and soil moisture loss. The following discussion will focus on soil cover as it affects soil moisture loss through evaporation.
Under undisturbed conditions, soil cover is predominantly composed of duff, litter, and stems of plants. Duff and litter layers form ideal surface mulches because they allow rainfall or snowmelt into the soil while blocking the escape of soil moisture through evaporation. They are also unfavorable seedbeds for weed seeds because duff and litter dry out quickly (Figure 5.26). Disturbed soils, on the other hand, are mostly composed of bare soil. Evaporation from the surface of bare soil can be high, extending to at least 6 inches below the soil surface, affecting seed germination and seedling establishment rates. Until roots of establishing seedlings have extended further into the soil profile, surface drying will negatively affect seedling establishment, especially on sites where water input and storage are already limiting.
Figure 5.26 — An effective mulch for seed cover is one that is stable and allows good airflow and rainfall entry, while reducing evaporation from the soil surface. |
![]() |
5.4.4.1 Soil Cover — How to Assess
Soil cover can be measured on undisturbed and disturbed reference sites or post-construction sites through ground cover monitoring protocols outlined in Section 12.2. In this protocol, the percentage of area in litter, duff, rock, vegetation, and bare soil is recorded and periodic measurements of litter and duff thickness are made. Transects can be conducted after disturbances. However, it is likely that where excavation has occurred, there will be no litter, duff, or vegetation cover present.
5.4.4.2 Mitigating for Low Soil Cover
Mulches for Seedlings and Cuttings — Mulches create a more favorable environment for establishing seedlings and cuttings not only by reducing surface evaporation, but also decreasing the amount of competing vegetation. There are two types of mulches for seedlings and cuttings — organic aggregate and sheet mulches. The organic aggregates are thickly applied ground wood or bark, while the sheet mulches are made from non-permeable or slightly permeable plastic, newspaper, or geotextile. Both types are placed around the base of the seedling and cover at least a radius of 1.5 feet from the base of the seedling.
Mulches for Sown Seeds — Selecting and applying mulches over sown seeds differs from those selected for planted seedlings and cuttings. Mulch application for seedlings and cuttings is typically too thick for seeds to germinate and grow through. An ideal seedbed mulch is one that is applied at the highest rates without affecting seedling emergence. Long-fibered mulches, such as straw, hay, or wood strands, create the greatest loft or thickness but, at lower rates, still allow light to penetrate and space for seedlings to emerge. Short-fibered mulches, such as wood fiber and paper found in hydromulch products, are more compact and create less loft. While these products reduce erosion rates, they are not necessarily good as seed covers.
5.5 Nutrient Cycling
Nutrient cycling is the process by which sites store and release essential nutrients for plant survival and growth. There are 13 elements, or mineral nutrients. Each fills a specific role or function in plant development and each possesses individual characteristics of movement and storage in the soil. This manual will not attempt to explain the role and function of each mineral nutrient (there are many good textbooks on this subject). It will instead focus on how nutrients cycle through vegetation and soils; how they are captured, stored and released; and what site components are essential to support these processes. In contrast to an agricultural system of managing optimum growth in crops through fertilization, the goal in wildlands revegetation is to create an environment that will support a self-sustaining native plant community that can develop through successional processes. This includes facilitating the establishment of nutrient cycles in a way that conserves, cycles, and builds nutrients in the system.
Mineral nutrients are stored in: 1) soil, 2) live or dead vegetation, and 3) rock. They are slowly released over time at varying rates. The rates at which nutrients are released from each source will determine their availability for plant uptake. Rock and fallen trees, for example, both hold essential nutrients, but release them to the soil at significantly differing rates. The fallen tree can take up to 100 years to decompose and release its nutrients; the weathering of rock might take over 100,000 years. Soil, on the other hand, can release nutrients in the order of weeks and months. Once released, these nutrients are stored, taken up by plants, lost through leaching or erosion, or reabsorbed in the soil.
On undisturbed sites, there is a dynamic exchange of nutrients throughout the year. Plants absorb nutrients from the soil through the root system and assimilate them into vegetative biomass. As plants, or portions of plants, die, they drop leaves, branches, and stems to the ground where they eventually decompose and return nutrients to the soil. The nutrients are stored in the soil and once again available for plant uptake. This natural process of nutrients moving from soil to vegetation and back again is referred to as nutrient cycling. The factors important in nutrient cycling are:
- Topsoil,
- Site organic matter,
- Soil carbon and nitrogen,
- Nutrients, and
- Soil pH and salinity.
In a healthy plant community, nutrients are constantly recycled with a minimum amount of nutrients lost from the site. On drastically disturbed sites, however, nutrient cycling functions poorly, if at all. The topsoil, which holds the greatest concentrations of available nutrients and supports the primary microbial activity on the site, is missing or mixed with the subsoil. Organic matter, which is a primary source of long-term nutrient supply and energy source, has also been removed. Soil nitrogen, the most critical nutrient for plant growth and site revegetation, is lacking. Soil nitrogen governs how quickly vegetation will return to a disturbed site and how much biomass it will ultimately support (Bloomfield and others 1982). Its availability is closely regulated by the amount of carbon present in the soil, which is also in flux on highly disturbed sites.
For many sites that lack topsoil, the subsoil in its place may have pH values that are higher or lower than the pH of the original topsoil. pH values at extreme ranges affect nutrient cycling by making many nutrients insoluble and unavailable for plant uptake. In addition, increased soil salinity, which can be caused by soil disturbance and amendments, can disrupt nutrient availability and provide unfavorable conditions for plant establishment.
5.5.1 Topsoil
Figure 5.27 — Topsoil is generally darker, more friable, and has more roots than subsoil. |
![]() |
The topsoil is the horizon directly below the litter layer that is characterized by high organic matter, abundant roots, healthy microbial activity, good infiltration rates, high porosity, high nutrient content, and high water-holding capacity (Jackson and others 1988; Claassen and Zasoski 1998). Most nutrient cycling takes place in the topsoil where the greatest biological activity occurs. Decomposing microorganisms flourish, feasting on dead vegetation and roots and releasing stored nutrients back to the soil. Most life forms occur in forest and range topsoils, including mammals, reptiles, amphibians, snails, earthworms, insects, nematodes, algae, fungi, viruses, bacteria, actinomycetes, and protozoa (Trappe and Bollen 1981). The top 6 inches of an acre of forest topsoil can contain as much as a ton of fungi and a half of a ton of bacteria and actinomycetes apiece (Bollen 1974). Topsoil depth is highly correlated with the nutritional status of the soil and, in Northwest forests, has been found to be highly correlated to site productivity (Steinbrenner 1981). Topsoils possess humus, which is what gives topsoils their dark color. Humus is a stable end product of decomposition, important for nutrient storage, soil structure, and water-holding capacity.
Sites lacking topsoil have significantly reduced productivity, and obtaining even minimal revegetation can be very difficult. Planted seedlings often fail or growth is significantly reduced, resulting in inadequate plant cover to protect the soils from erosion. Growth of planted trees can be reduced by one third to one half when planted in subsoil instead of topsoil (Youngberg 1981). Restoring these sites to functioning plant communities is unlikely without mitigating measures.
5.5.1.1 Topsoil — How to Assess
It does not take a soil scientist to determine the basic characteristics of topsoil and topsoil depth. It is simply a matter of digging holes and observing some of the basic topsoil characteristics. Usually topsoil can be differentiated from subsoil by simple observations. On undisturbed sites, for example, topsoil is visually differentiated from the underlying subsoil by having darker colors, less clay, better soil structure, and higher abundance of fine roots. In forest soils, topsoil depths can be more difficult to differentiate from subsoils because the color changes are not always distinct. Other attributes, such as the abundance of roots, lack of clays, increased soil structure, and lower bulk density, can be used instead. Section 5.11.1, Survey Topsoil, gives a general approach to surveying a site for topsoil.
5.5.1.2 Mitigating for Lack of Topsoil
Minimize Soil Disturbance — Where soils are especially fragile and reconstructing topsoil conditions is difficult, it is especially important to keep the "disturbance footprint" to a minimum (Claassen and others 1995). Sites with fragile soils include decomposed granitic soils, serpentine soils, and thin, high elevation soils.
Salvage and Reapply Topsoil — An effective practice in revegetating highly disturbed sites is salvaging and reapplying topsoils (See Section 10.1.4, Topsoil). This practice has been found to greatly increase plant growth and ground cover (Claassen and Zasoski 1994). Topsoil salvage and application requires good planning and implementation oversight. In the planning phase, a topsoil survey of the planned road corridor will identify the depth for topsoil removal; soil testing of the topsoil to be salvaged will indicate the nutrient status (See Section 5.11.1, Survey Topsoil). After topsoil is removed and stored, it is reapplied to the disturbed site, ideally at depths similar to pre-disturbance reference site topsoils.
Create Manufactured Topsoil — When topsoil is not available, "manufactured topsoil" can be created in situ or produced offsite and imported (See Section 10.1.4.5, Manufactured Topsoil). Manufactured topsoil will lack the native seed bank and some of the biological components of topsoil, but it can recreate a rooting zone high in nutrients and organic matter, with good water-holding capacity, porosity, and infiltration.
Create Planting Islands — If sources of manufactured or natural topsoil are scarce or too costly for broad scale applications, placing available topsoil in strategic locations, such as planting islands, can create a mosaic of productive growing sites.
5.5.2 Site Organic Matter
Site organic matter (OM) consists of plant materials in all stages of decomposition, including wood, bark, roots, branches, needles, leaves, duff, litter, and soil organisms. From a nutrient cycling standpoint, site organic matter assimilates nutrients drawn from the soil into live vegetation. Depending on the type of vegetation and the productivity of the site, the amount of nutrients held in organic matter can be significant. Figure 5.28 shows the quantities of four major nutrients held in the organic matter of a young Douglas-fir stand and Figure 5.29 shows the quantity of major nutrients found in the application of two inches of material derived from Douglas-fir and alder. These two examples show the possible nutrient reserves that organic matter can contribute to a disturbed site if they are used.
Figure 5.30 — Relative rates are a function of decomposition by Carbon to Nitrogen ratio and particle size. |
![]() |
While plants are essential in nutrient cycling, equally important are the decomposing organisms that release nutrients to the soil. Decomposers consist of thousands of specialized species of animals, insects, fungi, bacteria, and actinomycetes that survive on organic matter. Decomposing organisms not only release nutrients, but are also essential to the development of soil structure (See Section 5.3.3, Soil Structure).
On an undisturbed site, organic matter is in all stages of decomposition, from recently dead trees to soil humus, the end product of hundreds of years of decomposition. This understanding is important for restoring a site to a functioning plant community, since it is a reminder that nutrients are released throughout the life cycle of a plant community, not just at the beginning of the revegetation project. A site that has a range of organic matter in all stages of decomposition not only conserves nutrients but slowly meters them out over time.
The rate at which organic matter decomposes and releases nutrients is a function of: 1) soil to OM contact, 2) OM particle size, 3) carbon-to-nitrogen ratio (C:N), 4) temperature, and 5) moisture. Decomposition rates of organic matter are highest in soil because the greatest microbial activity occurs when soil is in direct contact with OM (Slick and Curtis 1985; Rose and others 1995). Organic matter placed on the surface of the soil as a mulch will decompose at a much slower rate than organic matter incorporated into the soil because there is less contact with the soil.
Organic matter particle size plays an important role in the rate of decomposition. Within the soil profile, the smaller-sized OM fractions decompose faster than the larger fractions due to greater surface area in contact with soil. Roots, leaves, needles, and very finely ground sawdust or bark often decompose at a much faster rate than larger materials, such as buried logs or large diameter branches. Materials with high carbon to nitrogen ratios (C:N), such as wood or bark, decompose at a much slower rate than materials with low C:N, such as green leaves and grass cuttings. As Figure 5.30 illustrates, high C:N organic matter will take much longer to decompose than low C:N material, but both will decompose faster when they are reduced in size.
Moisture and temperature also control decomposition rates; cold and dry environments have very slow rates compared to warm, moist sites.
5.5.2.1 Site Organic Matter — How to Assess
Duff and litter in reference, pre-, and post-disturbance sites can be measured through transects or plots (See Chapter 12). Estimating forest biomass of down, woody materials in different size classes can be done using photo series guides (Maxwell and Ward 1976a, 1976b, 1979). Fire specialists are experienced in estimating the amount of biomass in forested environments.
5.5.2.2 Mitigating for Lack of Site Organic Matter
Salvage and Reapply Litter and Duff — Duff and litter can store a significant amount of nutrients, especially on sites where layers are deep. It can be salvaged separately (See Section 10.1.3.11, Litter and Duff) or mixed together when topsoils are salvaged (See Section 10.1.4, Topsoil).
Chip and Apply Organic Matter — Road projects constructed through forested sites can generate high amounts of biomass that must be disposed, usually through burning. These materials can be a good source for slow-release nutrients. Methods of chipping and reapplication are discussed in Section 10.1.3. Chipped organic matter can be applied directly to the soil surface or mixed into the soil. High C:N organic materials, such as sawdust and bark, can be placed on the soil surface to prevent long-term nitrogen tieup in the soil, or they can be composted for several years to lower the C:N before adding as an amendment. Lower C:N materials, such as leaves, needles, and branches, can be placed in the soil with some addition of slow-release nitrogen to reduce the effects of nitrogen tie-up.
Apply Composts to Soil Surface — Compost functions as a soil cover to protect the soil from surface erosion while slowly decomposing and adding nutrients as the plant community develops. Decomposition rates of surface-applied compost are slower than mulch incorporated into the soil because there is very little soil contact. Nutrients are released at a slower rate and are available to the site longer. Leaving the soil surface very rough prior to the application of compost creates more soil-to-compost contact, which can increase the rate of decomposition of the compost.
Salvage and Place Large Wood — Large wood can be salvaged and placed in areas such as abandoned roads for microsite amelioration for revegetation. When placed in contact with the soil, large wood helps stabilize the surface of the soil from sheet and wind erosion, and can be used as buttresses to stabilize slopes. Seedlings planted on the north side of the logs can be protected from wind and sun during establishment. Large and small wood can be placed at the outlets of culverts as obstacles to capture and store sediments, reducing the amount of sediment reaching live streams (Burroughs and King 1989; Ketcheson and Megahan 1996).
Figure 5.31 — Placement of large wood adds long-term organic matter while creating microsites for planting seedlings. Large wood can also slow runoff and detain sediments from surface soil erosion. | ![]() |
5.5.3 Soil Nitrogen and Carbon
Nitrogen (N) is discussed separately from the other mineral nutrients because of its critical importance to plant growth and long-term development of plant communities. Carbon (C) is included in this discussion because of its unique relationship to nitrogen availability. Carbon governs the amount of available nitrogen in the soil while nitrogen regulates the rate at which carbon is broken down. Both factors play a critical role in microbiological activity and the development of soil properties.
Carbon to Nitrogen Ratio (C:N): Carbon is the energy source for soil microorganisms, and practically all site nitrogen eventually passes through these microorganisms (Woodmansee 1978). The rate at which carbon (or organic matter) decomposes is directly related to the amount of available nitrogen and the type of dominant microbes present in the soil. The greater the nitrogen, the greater the decomposition rates. If decomposing organisms do not find sufficient nitrogen in the organic matter, they will withdraw it from the soil, leaving little or no nitrogen for plant growth. This is usually a temporary condition, lasting several years. The tieup of nitrogen can greatly affect the establishment of vegetation if organic amendments, such as composts, wood chips, or straw, are incorporated into the soil without supplemental nitrogen.
With time, nitrogen is eventually released from the organic matter by microbial activity. This nitrogen, plus nitrogen released from dead and decomposing micro-organisms, becomes available for plant growth. As organic matter breaks down further, there becomes a net increase in available nitrogen. In the last stage of decomposition, microorganisms move to a steady rate of decomposition, releasing a constant nitrogen supply. This process can take several years or more depending on site factors and organic matter levels. C:N is an indicator of whether nitrogen will be limiting or in surplus. A C:N of 30:1 or greater indicates that decomposing organisms have consumed the available nitrogen in the soil, leaving little if any available nitrogen for plant growth. Plants respond by turning yellow and becoming stunted. A C:N below 18:1 is an indication that the decomposing organisms are releasing available nitrogen from the breakdown of organic matter at rates that exceed their need, thereby increasing nitrogen for plant uptake (Claassen 2006). For example, undisturbed topsoils typically have ratios of 10:1 to 12:1, which is sufficiently available for plant growth (Tisdale and Nelson 1975). Dry hay, on the other hand, has a C:N around 40:1, indicating nitrogen will probably be limiting if the hay is incorporated into the soil.
The use of high C:N materials is often discouraged because of concerns about tying up nitrogen. However, there are strategies where the use of high C:N ratio materials can aid the revegetation specialist in achieving project goals. One use of high C:N ratio materials can be incorporation into the top several inches of soil or application to the surface to intentionally tie up nitrogen. The applications would lower the availability of N to annual weedy species, which thrive on available nitrogen (See Section 5.8.1.2, Reducing or Eliminating Weed Growing Environments).
Soil Nitrogen Capital: Soil nitrogen capital can be categorized into three nitrogen pools, or reserves, based on its availability in the soil:
- Available nitrogen (referred to as "extractable nitrogen"),
- Slowly-available nitrogen (referred to as "mineralizable nitrogen"), and
- Unavailable nitrogen ("humified organic" or "fresh" forms).
Nitrogen capital can be viewed much like our banking system. Cash received from the bank teller is comparable to available nitrogen. When the money runs low, the teller replenishes it with money from the bank vault (similar to slowly-available nitrogen). Banks are backed up by money held in an extremely large banking reserve system, or unavailable nitrogen. While this money is not accessible, it is very important for the long-term stability of the banking system. Unavailable nitrogen is like the banking reserve system in that it backs up the nitrogen system and ultimately releases nitrogen to the plant community.
Like the banking reserve system, having high reserves of both slowly-available and unavailable nitrogen assures that available nitrogen levels will be released at constant rates over time, which is necessary for the development of a plant community. Figure 5.33 shows the relationship of different revegetation treatments on nitrogen capital.
On highly disturbed sites, all nitrogen reserves are low. The course of action in typical revegetation projects is to apply inorganic fertilizers during the seeding operation. While this immediately makes nitrogen available, it does little for increasing long-term nitrogen reserves. Within a year of application, most soils will need more available nitrogen to sustain plant growth. Organic fertilizers, on the other hand, provide a combination of available and slowly-available nitrogen. These fertilizers release nitrogen over several years, but are typically applied at rates not great enough to bring the nitrogen reserves up to levels for long-term plant community establishment (Claassen and Hogan 1998). Applying topsoil, composts, or low C:N organic matter into the soil are mitigation treatments that create the reserves of unavailable and slowly-available nitrogen important for a constant supply of available nitrogen over time.
Minimum Soil Nitrogen Levels: Total soil nitrogen is the sum of available, slowly-available, and unavailable nitrogen reserves. The level of total soil nitrogen varies by plant community and ecoregion. It can range from 20,000 lb/ac in deep forest soils of the Washington and Oregon coast (Heilman 1981) to as low as 800 lb/ac in desert grasslands of Southern New Mexico (Reeder and Sabey 1987). Shortgrass prairies in the Northeastern Colorado and shrub-steppe prairies of the Great Basin have a range of total nitrogen from 4,000 to 5,000 lb/ac (Reeder and Sabey 1987). Sites that are drastically disturbed often have nitrogen rates below 700 lb/ac. These sites cannot fully support vegetative cover. A minimum, or threshold, level of total soil nitrogen required for a self-sustaining ecosystem has been suggested at 625 lb/ac (Bradshaw and others 1982) to 670 lb/ac (Dancer and others 1977) for drastically disturbed sites. But Claassen and Hogan (1998) suggest much higher rates might be necessary. In their research on granitic soils in the Lake Tahoe area, they found a good relationship between total soil nitrogen and the percentage of plant cover. Sites with greater than 40% ground cover contained at least 1,100 lb/ac total soil nitrogen in the surface foot of soil. This implies that to maintain a minimum of 40% plant cover, sites like these must contain at least 1,100 lb/ac of nitrogen, with higher nitrogen levels necessary for higher plant cover (See Figure 5.34).
Target levels for available nitrogen released annually from nitrogen sources for plant growth range from below 27 to 50 lb/ac (Munshower 1994). These are nitrogen levels that should be considered when calculating fertilizer rates (See Section 10.1.1.6, Determine Fertilizer Application Rates).
5.5.3.1 Soil Nitrogen and Carbon — How to Assess
Nitrogen Testing — Soil testing for nitrogen should be conducted for: 1) topsoils that will be salvaged; 2) reference sites; and 3) post-construction soil materials. Procedures for collecting soil samples are presented in Inset 5.2, Soil Testing. The following nitrogen tests are available:
Total nitrogen: Total nitrogen is an important test to request; the results will be used to determine nitrogen thresholds and nitrogen amendment needs. The common total nitrogen tests are Leco and Kjeldahl. Total nitrogen has been found to correlate well with plant cover (Claassen and Hogan 1998).
Mineralizable nitrogen: This test requires the soil samples to be incubated for a period of time and then tested for available nitrogen. The results indicate the amount of slowly-available nitrogen present in the sample. It is a very good test to perform because the results correlate well with expected plant cover (Claassen and Hogan 1998). There are several types of incubation tests, so it is good to confer with the soil laboratory as to which tests would be most appropriate.
Extractable nitrogen: This test is less meaningful, since it only indicates available nitrogen, not what is in reserve. This test is often included in a soil testing package. Extractable N pool has the lowest correlation to the amount of plant cover growing on a site (Claassen and Hogan 2002). The most common test for extractable nitrogen is 2N KCl extract.
Nitrogen testing for composts and organic matter should be done by laboratories specializing in these tests. These laboratories should follow the testing procedures outlined in the Test Methods of the Examination of Compost and Composting (TMECC) explained in Section 10.1.3, Mulches and Section 10.1.5, Organic Matter Amendments.
Nitrogen Analysis — Soils laboratories report nitrogen in a variety of units, such as gr/l, ppm, mg/kg, ug/g, and percent. Unless these values are converted to pounds per acre, it is difficult to determine rates of fertilizer, compost, or topsoil necessary to restore site nitrogen. Use Figure 5.35 to convert lab values to total pounds per acre of nitrogen. These calculations account for soil bulk density, soil thickness, and coarse fragment content, which affect the total nitrogen levels of a site.
Nitrogen Thresholds and Deficits — Each plant community has a total nitrogen requirement that must be met in order to develop into functioning and self-sustaining systems. For successful revegetation efforts, a practical goal is to meet the minimum target, or threshold level, for total nitrogen. Threshold values, however, are not found in textbooks and must be developed from soil tests of disturbed and undisturbed reference sites. Conducting nitrogen tests on disturbed reference sites where revegetation efforts have succeeded, as well as reference sites where revegetation efforts have failed, can lead to determining a threshold value (Figure 5.34). Conducting soil tests on undisturbed reference sites, on the other hand, will define the optimum nitrogen levels and also bracket target nitrogen levels. Converting soil test results into total nitrogen per acre is shown in Figure 5.35, line E.
Post construction soils are typically deficient in nitrogen. In order to develop a strategy for bringing soil nitrogen above threshold levels, it is important to determine the approximate nitrogen deficit. Figure 5.35 shows how this can be calculated by subtracting the total nitrogen value of post-construction soils (Line E) from the threshold nitrogen value (Line F).
Carbon Analysis — Carbon is determined directly using the combustion method (Leco instrument) or indirectly with the Walkley-Black and/or loss-on-ignition methods. The Walkley-Black and loss-on-ignition methods measure the percentage of organic matter that can be converted to the percentage of carbon by multiplying by a lab factor. Since carbon makes up between 50% to 58% of the organic matter (Tisdale and Nelson 1975), most labs will use a conversion factor within this range. For all tests, it is important to remember that soil labs sieve out any materials greater than 2 mm. Prior to sending the sample to the lab, sieve out the rock fraction but leave any organic matter in the sample. Request that the lab not sieve the sample so that the results report out all carbon and nitrogen.
C:N — C:N is calculated by dividing % carbon by % nitrogen from the laboratory results obtained for nitrogen and carbon tests.
Figure 5.35 — Calculating the nitrogen deficit of a site — an example. |
![]() |
5.5.3.2 Mitigating for Low Soil Nitrogen
Develop a Strategy — It is important to develop a strategy for increasing nitrogen over time. It is not enough to just add fertilizer at seeding and call it good. On sites that are deficient in nitrogen, a strategy must be developed that considers the accumulation of nitrogen over time by all available sources — topsoil, mulch, compost, fertilizers, and nitrogen-fixing plants. Figure 5.36 shows an example of a strategy for increasing total soil nitrogen to a threshold level.
Topsoil — Salvaging and reapplying topsoil is an excellent way to increase total soil nitrogen on drastically disturbed sites. The depth to apply topsoil should be similar to the soil depth found in undisturbed reference sites or pre-construction soils. If topsoil material is limited, then using the calculations shown in Figure 5.35 can help determine the minimum depths to apply topsoil. Section 10.1.4, Topsoil discusses methods to salvage and apply topsoil. To determine if there is, or could be, a tieup or surplus of nitrogen in the salvaged topsoil, soil tests should be conducted and evaluated for C:N. C:N greater than 25:1 could benefit from the addition of nitrogen, while a ratio less than 8:1 will have available nitrogen for plant growth.
Composts — Applied on the soil surface and incorporated, composts can supply sufficient soil nitrogen for long-term site needs. Application rates for composts can be calculated using methods shown in Figure 5.35. Testing and application methods for compost are discussed in Section 10.1.3, Mulches and Section 10.1.5, Organic Matter Amendments.
Nitrogen-Fixing Plants — Significant quantities of nitrogen can be supplied by nitrogen-fixing plants (See Section 10.1.7, Beneficial Soil Microorganisms). Establishing nitrogen-fixing plants is a means of meeting short-term goals without having to amend with nitrogen fertilizers, and long-term goals by increasing the total nitrogen on the site.
Fertilizers — Applying nitrogen-based fertilizers to drastically disturbed soils is another means of increasing nitrogen levels, but it requires an understanding of fertilizers (composition and release), how the soils will capture and store nutrients, and how plants will respond to increased levels of available nitrogen. As the calculations in Figure 5.35 demonstrate, nitrogen based fertilizers cannot deliver enough nitrogen in one application for long-term site recovery of drastically disturbed sites. However, applied judiciously within an overall nitrogen strategy using topsoil, composts, and nitrogen-fixing plants, nitrogen-based fertilizers can be an effective tool in site recovery.
Not all sites or conditions require fertilizers. It might not be necessary to fertilize soils that have low C:N with nitrogen levels exceeding a minimum threshold level. In fact, applying fast release fertilizers may be a disadvantage on some sites by favoring weedy annuals over perennial species. A discussion on selecting fertilizers, calculating application rates, and determining methods of application are discussed in Section 10.1.1, Fertilizers.
Biosolids — Biosolids are rich in nitrogen and, if sources are available nearby, are a good means of raising soil nitrogen.
5.5.4 Nutrients
This section will broadly discuss the remaining mineral nutrients essential for plant growth (Figure 5.37). There are many references devoted to the role of nutrients in plant nutrition and the reader is directed to these sources for a more detailed discussion of each nutrient (Tisdale and Nelson 1975; Thorup 1984; van den Driessche 1990; Munshower 1994; Havlin and others 1999; Claassen 2006). The majority of research in soil fertility and mineral nutrients was conducted with the agricultural objective of optimizing crop yields through annual inputs of fertilizers, soil amendments, and irrigation. The primary objective of wildland restoration, however, is to restore or re-create self-sustaining plant communities that, once established, require very little input of resources. Unlike agriculture, revegetation of highly disturbed sites is not approached with the objective of optimizing the site for a single, high-yielding crop, but to develop a system of interrelated species that have evolved and adapted to site conditions. In wildland restoration, it is more important to re-create the components of the local soils than to change nutrient status based on agricultural models. For example, from an agricultural perspective, a serpentine soil has an imbalance of calcium and magnesium. Unless fertilizers containing a "correct" ratio of calcium and magnesium are applied to adjust this imbalance, the soils will be unsuitable for crop species. In wildland restoration, the approach is guided by the nutrient needs of the species endemic to the site, not to a generic agricultural crop. Since serpentine plant species have evolved on soils with these nutrient ratios, their nutrient requirements are vastly different than those of agricultural crops, or even native vegetation growing on adjacent, non-serpentine soils. In this example, the calcium to magnesium ratio would not be seen as an imbalance for native serpentine plant establishment, but as perhaps a "requirement" for certain endemic species to recolonize the site. This requires the revegetation specialist to compare post-construction mineral nutrient status to that of undisturbed or recovered reference sites to determine if there are deficiencies. Amendments can then be applied to bring nutrients and other soil factors to pre-disturbance levels or to levels that meet project revegetation objectives.
Success in wildland restoration is determined by its species richness, not biomass production, and being self-sustaining and resilient, not a system that requires constant energy inputs. By these standards, applying the basic agricultural model to wildland revegetation is limited.
5.5.4.1 Nutrients — How to Assess
The objective of nutrient analysis is to compare nutrient levels of post-construction, disturbed soils with those of pre-disturbance, or reference site, soils. Where there are large discrepancies, a strategy can be developed to bring low post-construction levels up to minimum nutrient levels. Since this is a comparative analysis, it is essential that the sampling, collection, and testing methods are identical.
Soil testing is a means of describing those soil characteristics that cannot be observed or accurately measured in the field. The tests include analysis of chemical properties including pH, soluble salts, macronutrients, micronutrients, and organic matter, and physical properties such as density, water-holding capacity, and texture. Soil testing is costly and if not sampled, analyzed and interpreted properly can lead to unneeded and expensive soil amendments and application practices. In many respects, it is better not to test soils than to test them or interpret the results incorrectly. Soil testing is performed with site survey, topsoil recovery, and reference site surveys (discussed in other sections) to 1) identify soil physical and chemical factors that will limit plant growth, 2) develop site-specific soil quality targets and 3) develop a set of revegetation treatments that will increase short and long term soil productivity targets. There are three components of soil testing — 1) soil sampling, 2) lab analysis, and 3) interpretation of lab results. Adhering to an established protocol for each component of soil testing is critical for developing appropriate revegetation treatments. Sampling soil — Soil sampling is the field collection of soils in a manner that best represents the soils of an area. The number of soil samples taken within a project area is usually kept to a minimum because of the expense of collecting and handling the samples and the cost of laboratory analysis. Taking a small amount of samples to describe a project site can be misleading, especially if the soils are extremely variable. This leaves the revegetation specialist with the challenge of determining the best approach to collecting soil samples in a way that most accurately represents the sites being described. The following guidelines are useful in developing a sampling strategy for soil testing: Determine the area to be sampled — The areas to be sampled are called sampling areas and they are typically revegetation units, topsoil stockpiles or topsoil salvage areas, or reference sites. For most projects, sampling areas will have no more than one sample collected from each, therefore the selection of the site to collect soils samples (the collection site) must be representative of the sampling area. The sampling area and collection site are not always one and the same. Only for small sampling areas, such as topsoil stockpiles or reference sites, will the collection site be the same as the sampling area. For larger sampling areas, such as revegetation units or topsoil salvage areas, the collection site will be a smaller, representative areas within the sampling area. Collect multiple subsamples — Once a collection site has been identified, a set of subsamples are collected. Collecting soil from one point is never enough! The number of subsamples to collect within a collection site should be based on the site's variability. Small collection sites generally require fewer subsamples than larger areas because there should be less variability. Undisturbed sites are typically more homogenous than disturbed sites and therefore require fewer subsamples. Guidelines for the numbers of subsamples to collect range from 6 for very homogenous sites to 35 for large, heterogenous sites. Randomly collect subsamples — Subsamples should be collected randomly within the collection site. For small areas like reference sites or stockpiled soil, the samples can be collected on a grid system. For very large areas, samples can be collected in a zigzag or "W" pattern at predetermined intervals. Determine sampling depth — Review the objectives for soil sampling and determine the sampling depth. If the objective is to characterize the soil for topsoil recovery, then the soil samples must be collected only in the topsoil horizon, in which case the depth of the topsoil would have to be determined prior to sampling. If it is known that the surface soil including duff and litter will be removed to 15" during topsoil salvage, then collection depth would be a sample 15" deep that included the duff and litter layers. If soil sampling objectives are to determine the nutrient and mycorrhizal status of a topsoil pile, the entire pile is the sampling zone and the subsamples must be collected from various depths within the piles as well as around the pile to obtain a representative sample. (If the surveyor felt that the interior of the pile was significantly different in nutrient status or mycorrhizae, then the pile could be stratified into collection sites — the exterior of the pile and the interior — and sampled separately.) Sampling of the subsoil is important because this will give an indication during planning of how the disturbed site will appear after construction and before mitigation. Collect a representative slice of soil — It is important to evenly sample the predetermined depth of soil. For example if the sampling depth is 0 to 15 inches deep, then the entire section of soil must be equally sampled for each subsample. Mix subsamples — Place subsamples in a clean bucket and mix thoroughly. From the composite subsamples, remove the required amount of soil to send to the lab for analysis. Determine coarse fragment content — If the soils are high in coarse fragments, the samples can be sieved in the field using a 2mm sieve. This will reduce the amount of sample weight to haul out of the survey area and give an estimate of the coarse fragment content; otherwise, sieve back at the office prior to sending to the lab. If the samples are wet or moist, they will need to be air dried prior to sieving. Record the percent coarse fragment content for the sampling area which includes large and small coarse fragments. This information will be used later to modify the lab results. Selecting a lab — The criteria for selecting a soil lab is typically based on costs, turnaround time, analytical tests and consulting services. Most labs offer a general test package for under $50 per sample (2004 prices) and deliver the results within two weeks of receiving the soil samples. For an added fee, laboratories will interpret the results of the analysis. While these are important reasons for selecting a lab, the primary criteria for selecting a lab should be based on the quality of the testing facilities. A common assumption is that all labs are of similar quality in their analytical testing, and that if a group of labs were sent the same soil sample they would report similar results for most tests. This is not typically the case, as several university reviews of laboratories have shown (Neufeld and Davison 2000; Rose 2004). In one comparison, eight reputable laboratories reported widely differing results for all soil nutrients when sent identical soil samples (Rose 2004). One reason for the variation in results is that there are usually several testing procedures that can be used to quantify a soil parameter. Some methods have greater accuracy and precision than others. The soil testing industry at this time has not settled on an agreed upon set of analytical methods to use. Even when the same tests are performed, labs often report different levels of accuracy (Rose 2004). Soil laboratories can voluntarily participate in a testing program called the North American Proficiency Testing (NAPT) program that will assess the quality of their analytical procedures. In this program, NAPT periodically sends all participating labs identical soil samples. Each lab analyzes the samples for mineral nutrients using established analytical procedures, then sends the results back to NAPT. The results from all labs are compiled and analyzed statistically and each lab is sent a report on how their results compared to the other participating labs. NAPT suggest that the accuracy be less than 10% of industry values and precision no greater than 15% of industry values (Neufeld and Davison 2000). These reports are not available to the public but laboratories might share them if asked. NAPT is not a certification program but is often a basis for a soil lab quality control program. The following is a checklist for selecting a high-quality lab (modified from Neufeld and Davison 2000):
If you receive no for the answers to any of these questions, another soil testing facility should be considered. If the selection is down to a couple of labs, consider sending duplicate soil samples with known properties ("checks") to each lab and compare the results using the NAPT suggested standards for accuracy and precision. Soil "checks" can be purchased through a proficiency testing program. Once a lab is selected, continue to ask for quality control reports. If the budget allows, periodically send duplicate "check" soil samples with your regular soil samples to assess accuracy and precision. |
![]() |
Nutrient testing should be performed on: 1) salvaged topsoils, 2) reference sites, 3) post-construction soil materials, and 4) amended soils. A guide to sampling soils for nutrient analysis is presented in Inset 5.2. Nutrient testing should evaluate total soil nutrient levels (long-term nutrient availability) as well as available levels (immediately available nutrients). Table 5.1 lists soil tests common to the western United States.
With soil laboratory results from reference sites and post-construction sites, determine which nutrients, if any, are deficient using a process outlined in Section 10.1.1.2, Develop Nutrient Thresholds and Determine Deficits. If a nutrient is found deficient, fertilizers, composts, topsoils, or other organic amendments can be applied to the soil to bring the nutrient above threshold levels. A process for determining fertilizer type, application rates, and application methods is presented in Section 10.1.1, Fertilizers.
5.5.4.2 Mitigating for Low Nutrients
Topsoil — Salvaging and reapplying topsoil is important for restoring nutrients to pre-construction levels, especially on sensitive soils (e.g., serpentine and granitic soils). The depth to apply topsoil should be at levels found in undisturbed reference sites or pre-construction soils, or can be calculated by methods described in Figure 10.25 in Section 10.1.4, Topsoil.
Compost — Incorporating composts is a good method for increasing nutrients to pre-disturbance levels. Determining which type of compost to select and how much to apply is discussed in Section 10.1.5, Organic Matter Amendments.
Table 5.1 — Common soil testing methods for the western United States (Horneck and others 1989; Munshower 1994; Teidemann and Lopez 2004). Note: Composts use a different set of tests due to the high organic matter (See Section 10.1.4, Topsoil and Section 10.1.5, Organic Matter Amendments). |
![]() |
Fertilizers — As discussed in Section 5.5.3, Soil Nitrogen and Carbon, fertilizers should be used within an overall nutrient strategy. See Section 10.1.1, Fertilizers, for a discussion on application methods, fertilizer types, timing, and other important aspects of fertilization.
Biosolids — Biosolids are rich in nutrients. If sources are available and transportation economical, this is a good way to add nutrients to disturbed sites.
5.5.5 pH and Salts
pH is the measurement of soil acidity or alkalinity, based on a logarithmic scale of 0 to 14. Soils with pH values below 7 are acidic, and those above 7 are basic. Basic soils have high amounts of bases (positively charged ions), such as calcium, magnesium, potassium, sodium, and phosphates. Basic soils have developed under arid and semi-arid climates and are found east of the Cascades and Sierra Mountains, throughout the Basin and Range province. Acidic soils have formed in wetter climates, where the continued movement of water through the soil profile has leached the bases from the soil. Acidic soils are found west of the Cascades and Sierra Mountains, and are most prevalent in the coastal ranges. Topsoils are typically more neutral when compared to underlying subsoil, whether the soils are acidic or basic. In some cases, the topsoil buffers the plant root systems from the underlying, inhospitable subsoil conditions. When topsoils are removed during construction, subsoils become the growing environment and, unless mitigating measures are taken, the results are disastrous for plant establishment.
Soil acidity or alkalinity, as measured by pH, affect mineral nutrient availability, mineral toxicity (Palmer 1990), and nitrogen fixation (Thorup 1984). In acid soils, the ability of plants to utilize many nutrients decreases, especially for calcium, and magnesium. As soil pH becomes more acid (less than 4.5), aluminum becomes more soluble and more toxic to plant growth. Low pH soils also hinder the establishment of nitrogen-fixing plants, such as legumes (Bloomfield and others 1982). Significant loss of rhizobia viability has been documented at pH levels less than 6 (Brown and others 1982).
Basic soils with pH values of 8.0 or greater indicate the presence of calcium carbonate (Thomas 1967). Calcium and magnesium are at such high levels that they can interfere with the uptake of other nutrients, notably phosphorus, iron, boron, copper, zinc (Campbell 1980). High pH soils typically have high salt levels, which can also restrict the growth of many plants. For example, as soil pH approaches 9, sodium concentrations become toxic to plants (Tisdale and Nelson 1975).
Soil salinity is the measure of the total amount of soluble salts in a soil. The term soluble salts refers to the inorganic soil constituents, or ions, that are dissolved in the soil water. The principal soluble salts in a soil contain the cations sodium, calcium, and magnesium, and the anions chloride, sulfate, and bicarbonate (Landis and Steinfeld 1990).
Almost all plants are susceptible to salt injury under certain conditions, with germinants and young seedlings being particularly susceptible to high salt levels (Figure 5.39). Soluble salts can injure plants in several ways:
- Reduced soil moisture. Salts can lower the free energy of water molecules, causing an osmotic effect and thereby reducing the moisture availability to plants.
- Reduced soil permeability. High salt concentrations (specifically sodium salts) can change the soil structure by reducing the attraction of soil particles, causing them to disperse. Pore space is lost and air and water movement within the soil profile are restricted.
- Direct toxicity. High levels of certain ions, including sodium, chloride, and boron, can injure plant tissue directly.
- Altering nutrient availability. Certain nutrients as salts can change the availability and utilization of other plant nutrients (Landis 1981; Landis and Steinfeld 1990).
High salt levels can be found in arid climates where there is inadequate rainfall to leach salts out of the plant root zone. Topsoils tend to be lower in salts than subsoils, so during construction when topsoil is removed, the resulting soils can be very high in salts and pH. In addition, high salt concentrations can be created by poor soil drainage resulting from compaction; when excessive amounts of fertilizer, manure, or compost are applied; or when de-icing chemicals applied to roads run off and enter the soil (Parent and Koenig 2003).
5.5.5.1 pH and Salts — How to Assess
The pH and soluble salts tests are standard analytical measurements that should be run for all soil samples sent for nutrient analysis. pH tests should also be run on soil organic matter amendments considered for mulch or incorporation into the soil. These tests are accurate, with pH results differing between laboratories by 0.1 to 0.2 points (Thomas 1967). pH and soluble salts can also be tested by the revegetation specialist on site or back at the office using reasonably priced equipment. When considering purchasing a portable pH meter, be sure that it will also measure soluble salts and that it has a tip that can be submerged easily in a soil slurry.
The most accurate method of assessing pH and salinity is through lab analysis. However, quick, reliable estimates can be made with a hand-held pH/electrical conductivity meter using the Saturated Media Extract (SME) method for preparing samples (Figure 5.38). This method requires a small amount of soil (50 cc) be placed in a jar. Just enough distilled water is stirred into the soil to make the surface "glisten" but not readily flow. The mixture is allowed to stand for approximately 15 minutes. If the probe can be inserted into the soil so that the sensors are completely covered, then a pH and conductivity reading can be directly made. If the probe can not be submerged in the slurry, then a larger sample of soil or a gravity or vacuum filtration system solution should be used to extract enough water to test.
Figure 5.39 — Soluble salts will injure germinants and, at high concentrations, can damage established plants. (Values are based on the SME method of conductivity measurement.) |
![]() |
5.5.5.2 Mitigating for Low pH Soils
Apply Liming Materials — Raising the pH through the application of liming materials is a common agricultural practice that can be applied to revegetating road sites (See Section 10.1.6, Lime Amendments).
Apply Appropriate Fertilizers — Some commercial fertilizers, especially ammonium-based fertilizers such as ammonium nitrate, ammonium sulfate, and ammonium phosphate, will reduce pH (Havlin and others 1999) and should be limited on acidic soils. Fertilizers that have calcium, magnesium, or potassium in the formula are more appropriate for low pH soils. Examples of these fertilizers are calcium nitrate, potassium nitrate, magnesium sulfate.
Apply Lime With Organic Matter — Incorporation of lime will lower pH. On acid soils, application of lime with organic matter will raise the pH of the soil (See Section 10.1.6, Lime Amendments).
Apply Topsoil — Where topsoils have been removed leaving very basic or very acidic subsoils, reapplying topsoil or manufactured topsoil can moderate pH levels.
5.5.5.3 Mitigating for High pH Soils
Apply Sulfur — Agricultural soils can be treated with sulfur to lower pH, but high quantities of sulfur and irrigation must be applied to lower the pH just slightly (Havlin 1999). The use of sulfur in wildland revegetation therefore is not a widely practiced method.
Irrigation — Applying irrigation water is another method of reducing soil pH by leaching out bases. However, the amount of water needed to lower pH levels can be very high; in most cases, using irrigation is not a viable mitigating measure. It is also difficult to find irrigation water in arid environments that is low in bases and salts. Applying irrigation water high in bases will raise pH and salt levels in the soil, compounding the problem.
Apply Organic Matter — Incorporated composts or other types of organic matter can lower soil pH as the organic matter decomposes (Havlin and others 1999). For arid sites, however, the pH and conductivity of the organic matter must be tested prior to purchase to avoid the possibility of introducing organic matter high in salts.
Add Nutrients — To compensate for the tie-up of certain nutrients, the addition of nutrients through fertilization might be considered. The benefits of using fertilizers on arid soil must be offset by the possibility of creating fertilizer salt problems.
5.5.5.4 Mitigating for Salts
Since high levels of soluble salts are usually caused by poor soil management, the key to mitigating high salinity is to avoid creating the conditions that could potentially cause those levels. In soils where internal drainage is poor, prevention may be the only feasible approach for reducing salt problems.
Avoid Mulch or Soil Amendments With High Salinity — Testing all materials to be applied to the site will aid in the prevention of increased salt levels in the soil. Amendment materials with electrical conductivity readings in excess of 1,000 mS/cm should be avoided.
Reduce Commercial Fertilizers — Some commercial fertilizers, control-release fertilizers (CRF) in particular, can significantly increase the soluble salts found in the soil. This can be a major problem when using CRF in arid conditions. The fertilizer will begin to release following wet, warm spring conditions, but will not be leached through the soil without significant rainfall through the summer. Salts can build up to damaging levels, both on the surface and in the plant root zone.
Apply Gypsum With Irrigation — Incorporation of gypsum (calcium sulfate) followed by leaching can be effective in situation where sodium is the cause of high soluble salts (e.g., de-icing materials have been applied to roads). The calcium in gypsum will displace sodium and the sodium will then leach out of the soil profile with irrigation or rainfall (UMES 2004).
Irrigation — Application of irrigation water could potentially leach the salts from the soil. The amount of water depends on the soil type. In arid soils, application of 6 inches of water can reduce salinity levels by 50%; 12 inches can reduce by 80%; and 24 inches can reduce by 90% (UMES 2004). Application of irrigation water would need to take place over several days. However, for most sites, this is not practical due to high costs.
5.6 Surface Stability
Surface stability is the tendency of the soil to remain in place under the erosive forces of rain, wind, and gravity. Good surface stability is essential for establishing plants, reducing erosion, and maintaining high water quality. If the soil surface is unstable, seeds will wash or blow away. They can also move downslope through freeze-thaw or gravity before they can germinate. The same processes that stabilize seeds will also stabilize the soil surface. As soil is removed, soil productivity is reduced. With excessive erosion, plant roots are exposed, increasing the possibility of seedling mortality.
Site factors that influence surface stability and soil erosion are:
- Rainfall and wind,
- Freeze-thaw,
- Soil cover,
- Surface strength,
- Infiltration rates,
- Slope gradients,
- Surface roughness, and
- Slope lengths.
All surface erosional processes start first with the detachment of soil through the forces of rainfall, wind, or frost heave. These forces loosen seeds and soil, making them more susceptible to transportation off the site. Water moving over the surface of the soil during rainstorm events is the primary factor in moving seeds and soil into stream channels, resulting in lost seeds and water quality problems. This occurs when infiltration rates (the rate water moves through the soil surface) are lower than rainfall rates. If slope gradients are steep, slope lengths are long, or surface roughness is low, surface water picks up energy and transports greater and greater amounts of soil and seeds downslope. As this energy increases, eventually water becomes concentrated with force to cut through the surface of the soil, creating rills and gullies. The degree to which soils detach is directly related the percentage of soil cover protecting the soil (more cover, less erosion) and to the soil strength, or the capacity for individual soil particles to hold together under erosional forces. The result of soil erosion can often be detected on road cuts by noting how much sediment is in the ditch line. If the ditch is full of recently deposited sediment (recently deposited sediment usually lacks vegetation), there is a good chance that sediment came from the cut slope. An inspection of the surface of the cut slope will indicate if the sediment originated there. Gravels, cobbles, and even small plants will show the results of soil movement.
5.6.1 Rainfall and Wind
Each site has unique rainfall and wind patterns that will affect the stability of the soil surface. High rainfall intensities and high winds can move seeds and soil particles off-site through erosional processes. In water erosion, raindrops begin the erosion process. Raindrops have been likened to small bombs. In heavy rainstorms, they fall with such force (possibly between 15 and 20 miles per hour) that, when they hit the soil surface, they create an impact that can blast the soil or seeds several feet away. Raindrops leave behind small craters that are compacted and sealed with fine soil particles, reducing infiltration rates.
The intensity of rainfall determines how much soil is detached. A high intensity rainfall will detach more soil particles than a low intensity rainfall. But detachment is only one aspect of erosion; it takes surface runoff to move soil and seeds downslope. If an intense rainstorm lasts only a short period of time, there will be insufficient water to exceed infiltration rates and water will absorb into the soil. If the duration is long, some water will not enter the soil, but will run over the surface carrying soil and seeds downslope. The most critical weather events, therefore, are those that bring high intensity rainstorms of long duration. These typically occur during major summer thunderstorms or Pacific winter storm systems. Precipitation in the form of snow is not typically a problem for surface erosion. Snow protects the soil surface from rainfall splash, and snowmelt usually occurs at such slow rates that even soils with low infiltration can absorb the water.
When projects are located in areas of high winds, surface soils will be susceptible to wind erosion. Wind forces will detach soil particles and seeds and move them offsite. In extreme conditions, high winds can remove several inches of surface soil, exposing roots (Figure 5.40).
5.6.1.1 Rainfall and Wind — How to Assess
It is unlikely that climate reports or weather records will give the duration and intensities of rainfall events for site level planning. Digital rainfall gauges are available that record the amount of rainfall and time it occurred. This information is used to determine duration and intensity. While the cost of this equipment is high, it is becoming more affordable. There are many types of digital rain gauges available, ranging in price and quality. It is important to select a digital rain gauge that is rugged, self maintaining, and can record for long periods of time.
Permanent wind speed equipment is available, but most likely beyond the reach of most project budgets. Site visits during different times of the year give some indication if wind is a problem. Site characteristics, such as position on slope (ridgelines are more prone to wind than valley floor) or proximity to forested environments (forests often reduce wind speeds) can be used to infer wind forces. Vegetation can also indicate the effect of wind. For example, prevailing winds can be determined from the growth habits of trees and shrubs on site. Local residents may also provide some information on local weather events.
Assessing the risk of wind erosion can be determined by knowing soil texture. Those soils most prone to wind erosion are non-cohesive soils, ranging from silts to coarse sands. Clay soils (cohesive soils) and very rocky soils are far less prone to wind erosion.
Figure 5.41 — Continual freeze-thaw conditions can push root systems of planted seedlings out of the ground, reducing growth and potentially killing seedlings. | ![]() |
5.6.1.2 Mitigating for High Rainfall and Wind
Minimize Disturbance — In areas of high rainfall or sites where water quality values are high (near streams or rivers), the best engineering design is to keep the footprint of the construction project disturbance to a minimum. Not only does this reduce the risk of delivering sediment to the aquatic system, it can reduce project costs by reducing the amount of area needing revegetation.
Integrate Erosion Practices — In disturbed sites, especially those near streams, the integration of erosion practices with plant establishment techniques offers the best approach to stabilizing the soil surface. These include practices such as increasing soil cover, shortening slopes, reducing slope gradients, leaving roughened surfaces, increasing infiltration rates, and quickly establishing native vegetative cover. These will be covered in the following sections.
Use Appropriate Mulching Practices —
Assessing the potential for high winds on the site should be considered when applying mulches because mulches can be removed from the soil surface under strong winds. Some materials, such as wood strand mulches (See Section
10.1.3.10, Wood Strands), have been tested under high wind conditions and, because of higher weights and interlocking particles, these materials are more resistant to high winds. Lighter mulch materials, such as straw and hay, are more susceptible to removal by winds.
On windy sites, straw and hay are often crimped into the soil, but this operation can also cause soil compaction. Tackifiers used with hydromulches are used effectively to stabilize straw and hay mulches. Mulches placed on roughened soil surfaces can also reduce the potential that the mulches will be lost by wind.
Place Large Woody Material — Down woody material such as trees and large branches can bock the soil surface from wind and rainfall.
5.6.2 Freeze-Thaw
Freeze-thaw is the process of ice formation and ice melting that occurs within the surface of the soil. In this daily cycle, temperatures drop at the soil surface at night and water begins to freeze within the soil pores, creating ice crystals. As ice crystals continue to form, water is drawn from the soil below through capillary action to replace the water that created the ice crystals. During freezing, ice crystals expand in the soil and push soil aggregates apart (Ferrick and Gatto 2004), weakening the internal structure of the soil. When soils thaw during the day, soil strength has been greatly reduced (Gatto and others 2004), leaving the soil surface significantly less resistant to erosional forces. Freeze-thaw is considered one of the least understood aspects of soil erosion (Ferrick and Gatto 2004) and yet accounts for significant annual soil losses (Froese and others 1999).
The formation of ice crystals will destabilize the seed germination environment. Freeze-thaw cycles affect germinating seeds by creating ice crystals that physically push the germinating seeds above the soil surface, exposing the emerging roots to extremely harsh conditions for seedling establishment, including low humidity, high temperatures, and sunlight. On steeper slopes, soil particles and germinating seeds will move downslope after each freeze-thaw cycle, further destabilizing the seed germination environment. Freeze-thaw processes can also affect seedling establishment. Long periods of freeze-thaw cycles can push container seedlings out of the ground, exposing roots and, in many cases, killing seedlings (Figure 5.41).
Soils most susceptible to freeze-thaw effects are those: 1) with a high silt content (or compacted sands), 2) with an adequate supply of soil moisture, and 3) that are cold. Silt-sized particles have pore sizes that are small enough to pull moisture to the freezing front through capillary action, yet large enough to form ice crystals (Ballard 1981). Soils with coarse textures, like sands, do not provide good capillary rise because the pores are too big; fine-textured soils (high in clay) have good capillary characteristics, yet do not have large enough pores for ice crystals to form (Ferrick and Gatto 2004). Sands have been reported to be frost-susceptible when they are compacted because the size of the pores is reduced, encouraging capillary rise (Gatto and others 2004). Soil cover, which includes litter, duff, and organic mulches, do not typically have good capillary rise characteristics, and therefore are less frost-susceptible. In addition, soil cover offers good thermal protection, which moderates the degree of freezing and thawing at the soil surface. The effects of freeze-thaw on surface stability increases as slope gradients steepen; the steeper the slopes, the greater the movement of seeds and soil downslope each day. Consider a seed or soil particle on a steep slope that experiences a 2 inch rise on top of an ice crystal (Figure 5.42); when the crystal melts, the seed or soil particle drops to a different point further downslope. After many freeze-thaw cycles, the distance traveled by the seed or soil particle can be significant.
5.6.2.1 Freeze-Thaw — How to Assess
Freeze-thaw processes occur typically in the spring and fall, when soil moisture levels are high and soil temperatures are cold. Soil surfaces that have undergone freeze-thaw cycles will have a very loose crust that will collapse when touched or walked upon. Gravels are often perched on pedestals, but give way under light pressure.
Project areas with bare surface soils high in silts should be considered prone to freeze-thaw processes in the spring or fall. Compacted soils high in sands are also susceptible. Soils with deep mulch or litter layers are less susceptible.
5.6.2.2 Mitigating for Freeze-Thaw
Apply mulch. Available research on the mitigation of freeze-thaw effects is slim, but it can be assumed that practices that insulate the soil surface and increase pore sizes will reduce some of the effects of freeze-thaw. The addition of organic mulches will provide insulation. The deeper the mulch layer, the less propensity for freeze-thaw at the surface. Hydromulch applications at typical rates of 1,000 to 2,000 lb/ac are not deep enough to moderate surface temperatures, or strong enough to resist the destabilizing effects of ice crystal formation on surface strength. Mulch applications that are too deep will bury the seeds, and seedlings will not emerge. In this case, using mulches such as pine needles and wood strands are options because application can occur at greater thickness but still allow light through for seedling emergence. If ice crystals do form and push newly emerged seedlings from the ground, these types of mulches will protect portions of exposed roots within the mulch layer from drying out, while also preventing the lifted soils from moving downslope by gravity (See Section 10.1.3.10, Wood Strands and Section 10.1.3.11, Litter and Duff). For planted seedlings, the application of very deep mulches should reduce the effects of freeze-thaw.
Till Compacted Soils — Sandy soils are very susceptible to freeze-thaw if they are compacted. Loosening soils through tillage is perhaps the best method of mitigating the effects of freeze-thaw on these soil textures (See Section 10.1.2, Tillage).
Avoid Wet Soils — Planting or sowing in soils with high water tables and poorly draining soils should be avoided. The extra moisture in these soils will feed ice crystal formation, creating a risk to establishing seedlings.
Maintain Some Overstory Canopy — Trees and shrubs will moderate surrounding temperatures, reducing the potential for freeze-thaw.
5.6.3 Soil Cover
Soil cover is the layer directly above the surface of the soil. In an undisturbed environment, soil cover is composed of duff, litter, live plants, and rock. Soil cover is very important for surface stability because it dissipates energy from rain drop impact before it reaches the surface of the soil. A good cover can protect the soil from high intensity rainfall events. Furthermore, if overland flow should occur during storm events, soil cover will slow the movement of runoff and capture sediments and seeds before they move downslope. Section 5.4, Water Loss, discusses how soil cover was important for reducing evaporation; this section will discuss its role in stabilizing the soil surface and reducing erosion.
When the soil surface lacks cover, it is subject to the direct forces of raindrop impact, overland flow, wind, and gravity. These forces not only move soil offsite, affecting water and air quality, but they also displace seeds or remove soil from around newly developing seedlings (Figure 5.43). A lack of soil cover will impact revegetation objectives by reducing the quantity of seeds that will germinate. Seeds that do germinate will be negatively affected by soil splash and sheet erosion that removes soil from around the seedling roots. The severity of soil erosion and seed movement is directly related to the percentage of bare soil.
After construction, most organic soil cover is removed. What remains is bare soil and coarse fragments (gravel, cobble, and stone). Left unprotected, bare soil will erode during rainstorms, leaving a pavement of gravel, cobble, and stone. If the amount of coarse fragments in the soil is high, then the percentage of the soil surface covered by coarse fragments will also be high. By the third year, erosion rates on unprotected bare soils typically fall to a tenth of the rate of the first year rate because of the formation of a soil cover of coarse fragments (Megahan 1974; Ketcheson and others 1999). While this process produces less sedimentation to stream systems, the high coarse fragments at the soil surface are not a good environment for seed germination. For this reason, it is important to quickly stabilize the soil surface after soil exposure; this is one of the reasons that road specifications call for temporary road stabilization during the construction period.
5.6.3.1 Soil Cover — How to Assess
Soil cover can be measured by establishing transects and recording the percentage of rock, vegetation, litter, duff, and bare soil. A monitoring protocol for measuring soil cover is presented in Chapter 12.
5.6.3.2 Mitigating For Low Soil Cover
The primary objective of most revegetation projects is to stabilize the soil surface and create an optimum environment for seeds to germinate and plants to establish. Initial surface stabilization must remain effective until plants become established and protect the surface from erosion through vegetative cover. Therefore, the selection of surface stabilization methods must be based on: 1) the effectiveness as a soil stabilizer, 2) the quality as a seed germination and plant establishment environment, and 3) longevity.
Apply Mulch — There are a variety of mulches with varying qualities and longevities which should be considered based on the erosional potential and revegetation needs of each site (See Section 10.1.3, Mulches). Short-fiber mulches, such as wood fiber and paper mulches, applied with a tackifier are very effective in protecting the soil from rainfall impact. However, after a year, these mulches are usually no longer effective. On many sites, protection for a year is adequate for establishing a good vegetative cover. On sites that are cold, arid, or semi-arid, the establishment of vegetative cover can take longer than one year (Figure 5.45). On these sites, soil surface protection will require longer-lasting mulches such as straw, pine needles, hay, wood chips, wood strands, or erosion fabrics.
5.6.4 Surface Strength
When soil cover is removed, the surface of the soil is exposed to the erosive forces of raindrop impact, surface flow, freeze—thaw, and wind. How strongly soil particles bind together determines how much soil particles are detached and moved by these forces. Topsoils with good aggregation and high organic matter will be more stable than subsoils or soils low in organic matter. Soils high in clays have greater strength than sands and silts, which lack cohesiveness. Seeds have no cohesive properties and, when sown on the surface of the soil without mulches or tackifiers, are very susceptible to erosive forces.
5.6.4.1 Surface Strength — How to Assess
Determining the soil texture of the surface soil is a simple way to determine soil strength (See Section 5.3.1, Soil Texture). Soils low in clays (<15%) and high in sands will have low surface strength (Figure 5.46). In most cases, soils lacking topsoil will have reduced surface strength due to the lack of roots and organic matter that hold the soil particles together. The rainfall simulator can also be used to determine soil strength because it measures the amount of sediment that is detached from surface soils under various rainfall intensities (See Section 5.6.5, Infiltration Rates). The USDA Natural Resources Conservation Service has developed a field test for determining surface stability for water erosion (Inset 5.3). This method rates how well surface soil samples maintain their stability after being agitated in water.
5.6.4.2 Mitigating For Low Surface Strength
Apply Tackifiers — Surface strength can be increased for up to a year or longer by applying tackifiers by themselves, with hydromulches over bare soil surfaces, or to bond straw onto the site (See Section 10.3.2, Hydroseeding). These products strengthen the bonds between surface soil particles. Seeds applied with a tackifier are held tightly to the soil surface, reducing the likelihood that seeds will be detached and moved.
Apply Mulch — Applying a long-fiber mulch to the soil surface can increase the overall surface strength because of the interlocking nature of the fibers (See Section 10.1.3, Mulches). The application of erosion mats can also increase surface strength.
5.6.5 Infiltration Rates
Infiltration is the ability of the soil surface to absorb water from rainfall, snowmelt, irrigation, or road drainage. A high infiltration rate indicates that the soil surface can transmit high rates of water input from sources such as a thunderstorm; a low rate indicates that the surface has low capability of absorbing water. When infiltration rates are lower than the rate of water input, runoff or overland flow will occur. Under these conditions, runoff can detach and transport soil particles, resulting in soil erosion and water quality problems. Overland flow can also remove sown seeds.
The size, abundance, and stability of soil surface pores determines the infiltration rates of a soil. Large stable pores created by worms, insects, and root channels will absorb water quickly and have high infiltration rates, while surfaces that have been compacted, topsoil removed, or are low in organic matter will have poor infiltration rates.
Under undisturbed conditions, infiltration rates are high, especially where a litter and duff cover exists. When soil cover is removed, the impact from raindrops can seal the soil surface, creating a crust that will significantly reduce infiltration rates. Infiltration rates are also reduced when the soil is compacted by heavy equipment traffic.
5.6.5.1 Infiltration Rates — How to Assess
The most accurate equipment for measuring infiltration rates is the rainfall simulator. This method is an attempt to create rainstorms of different intensities under controlled conditions and measure how the soil surface responds. Infiltration rates are determined when runoff occurs. Runoff water is measured at the bottom of the plot to calculate runoff rates and sediment yields (Figure 5.48). While most rainfall simulators were developed for agricultural operations, several have been developed specifically for wildland conditions. These simulators were built for transportability and conservation of water, since construction sites are often in remote locations and often far from water sources. The "drop-forming" rainfall simulator, developed for wildland use, delivers rainfall at the drop size or impact velocity determined for the climate of the project site (Grismer and Hogan 2004).
Using the rainfall simulator in revegetation planning is expensive, yet it is an important tool and should not be discounted because of the cost. Specifically, rainfall simulation used to compare the effects of different mitigating measures, such as mulches or tillage, on runoff and sediment production takes the guess work out of whether such measures are effective. This quantitative evaluation of erosion control methods might be essential in areas where water quality objectives are critical.
In most cases, infiltration rates are broadly estimated under routine field investigation by inference from site conditions. Infiltration rates can be considered high when the soil surface has not been disturbed and has a high percentage of cover. With compaction and loss of surface cover, infiltration rates are proportionally reduced. It is often assumed that construction activities that remove surface cover or disturb the topsoil will significantly reduce infiltration rates to levels that will create overland flow under most rainfall intensities.
5.6.5.2 Mitigating For Low Infiltration Rates
Tillage — Infiltration rates can be increased through soil tillage (See Section 10.1.2, Tillage) which reduces compaction, increases macro-pore space and creates surface roughness. Depending on the erosional characteristics of the site, the positive effects of tillage on infiltration rates might only remain effective through a series of rainfall events.
Incorporating Organic Matter — Incorporating organic matter into the soil surface can increase the longevity of infiltration rates created through tillage by forming stable macropores (See Section 10.1.5, Organic Matter Amendments). Unless macropores are interconnecting or continuous, however, they will not drain well (Claassen 2006). One method for creating continuous pores is to use long, slender organic material, such as shredded bark or hay. Compared to short organic materials, like wood chips, longer materials should increase infiltration rates. Incorporating higher quantities of organic matter will also increase porosity because of the potential of organic material to overlap and interconnect.
Surface Mulch — Applying surface mulch will reduce the effects of rainfall impact on surface sealing and reduce soil erosion rates (See Section 10.1.3, Mulches). It does not, however, necessarily increase infiltration rates. Studies have shown that, while sediment yield can be less with the application of mulches, runoff rates are not necessarily reduced (Hogan and Grismer 2007).
Plant Cover — The best long-term way to increase infiltration is to create conditions for a healthy vegetative cover. Good vegetative cover will produce soils with extensive root channels, aggregated soil particles, and good litter layers.
5.6.6 Slope Gradient
Slope angle or gradient is important in surface stability because it directly affects how soil particles will respond to erosional forces; the steeper the slope, the greater the erosional forces will act on the surface of the soil. Slope gradient is a dominant factor in water erosion and dry ravel erosional processes. In water erosion, the rate of soil loss and runoff from disturbed soil surfaces increases incrementally as slope gradient steepens. In dry ravel erosion, the surface remains stable until a specific slope gradient is reached, and then soil particles move downslope under the direct effects of gravity. This critical angle is called the angle of repose and it can be likened to the angle that accumulated sands make in an hourglass. Only non-cohesive soils, such as sandy, gravelly, or silt textured soils, have an angle of repose; soils with significant amounts of clays do not.
Dry ravel is occurring 24 hours a day. When you hear a piece of gravel or sand grain rolling down a cut slope, that is dry ravel. Not only does dry ravel create a constant supply of material to ditches, it also is impossible to revegetate unless the surface is stabilized. Seeds that germinate on steep, raveling slopes typically will not have enough time to put roots down deep enough to stabilize the surface before they roll downslope or are buried by soil particles rolling down from above. Figure 5.49 shows the angle of repose on a pumice sand parent material.
5.6.6.1 Slope Gradient — How to Assess
Slope gradient is quantified in several ways, as shown in Figure 5.50. For road construction projects, slope is usually expressed as the rise (vertical distance) over run (horizontal distance). A 1:3 road cut, for example, defines a slope that rises 1 foot over every 3 feet of distance. Biologists, including range, forest, and soil scientists, use percent slope as a measure of slope angle. This is calculated by measuring the number of feet rise over a 100 foot length. Slope gradient as expressed in degrees is not commonly used.
Slope gradient can be measured in the field using a handheld instrument called a clinometer. This equipment reads slope angle in percent slope and in degrees. Readings from a clinometer can be converted to rise over run notation using the chart in Figure 5.50. Road construction plans display the slope gradients for every cross-section corresponding to road station numbers (See Figure 3.3, in Section 3.2.3, Cross-Section View). Using these cross-sections, slope gradients can be identified on the plan map by color coding the run:rise for cut and fill slopes. For instance, 1:1 cut and fill slopes might be highlighted red for areas of concern, while those areas with gradients 1:3 or less might be light green, favorable areas for mitigation work. This exercise can quickly identify areas where the highest risk for soil erosion and seed movement might occur.
Slope angle plays a key role in revegetation planning because of its potential limitation on the types of mitigation measures that can be implemented. Figure 5.50 shows which practices are generally limited to gentle slopes and which can be implemented on steep slopes.
5.6.6.2 Mitigating for Steep Slope Gradients
Lengthen Cut or Fill Slopes — Slope gradient can be reduced by increasing the length of the disturbance. This will make the revegetation effort easier and more successful, but will increase the amount of area, or construction footprint, of the project.
Road planners consider many factors when they design the steepness of cuts and fills. A common design strategy is to create cut and fill slope gradients as steep as possible. This practice disturbs far less land, resulting in less area to revegetate. Because there is less exposed soil, the potential for soil erosion is considerably reduced. Furthermore, there is a substantial cost savings with less excavation and revegetation work. Figure 5.51 gives an example of how increasing the steepness of a cut slope on a typical 1V:2H natural ground slope substantially decreases the length of disturbed soils.
The main drawback of steepening slopes, however, is that steeper slopes are much harder to revegetate, and the selection of revegetation practices available are reduced as the steepness of the slope increases (Figure 5.50). On sites with 1V:5H slopes or less, all revegetation practices are possible; on slopes approaching a 1:1 slope, the threshold of most revegetation practices has been reached, as well as the limit of what can be successfully revegetated. It is important to work with the design engineers early in the planning stages to consider the effects of slope gradient on meeting revegetation objectives.
Create Steep, Hardened Structures — Creating hardened structures or walls, such as retaining walls, crib walls, gabion walls, or log terrace design structures at the base of the slope will allow gentler slope gradients to be constructed above the structure. The drawback to hardened structures is that most are hard to revegetate.
5.6.7 Surface Roughness
Slopes that have roughened surfaces trap water during the initial stages of the runoff processes (Darboux and Huang 2005). Roughened surfaces consist of micro-basins that capture and store soil particles and seeds that have become detached in the erosional processes. Seeds that have been transported short distances into these depressions are often buried by sediments. Moderate seed covering from transported soil can enhance germination as long as the seeds are not buried too deeply. Micro-basins can also be relatively stable during the period for seed germination and seedling establishment. Surface roughness also reduces the effects of wind by reducing wind speed at the soil surface. However, as the micro-basins fill up with sediments, they become less effective in capturing sediments and seeds.
5.6.7.1 Surface Roughness — How to Assess
There are many methods of measuring surface roughness which include paint and paper profiling, laser profiling, and pin boards. A simple field method for assessing surface roughness is using a cloth tape that conforms to the surface of the soil. In this method a tape is stretched a predetermined distance (e.g., 10 feet) and staked at both ends of the tape. Keeping the tape attached to one stake, the other end of the tape is released. Beginning at the staked end, the cloth tape is placed in contact with the soil surface and conforms to the micro-topography for the length of the tape. Tape distance is recorded where the end of the tape meets the end stake. The new distance is divided by the original distance to determine the surface area coefficient. The greater the distance between the two measurements, the greater the surface roughness.
5.6.7.2 Mitigating Measures For Smooth Soil Surfaces
Leave Surface Roughened — There is a tendency by many project engineers to "beautify" construction sites at the end of the project by smoothing soil surfaces. While basic landscape shaping is essential, the soil surface should be left as rough as possible. In many instances, leaving cut slopes "unfinished" or in the "clearing and grubbing" stage provides excellent seed bed diversity and growing environment. The diversity of micro-habitats provides greater climatic and soil environments for seed germination.
Surface Imprinting — Imprinting the soil surface to create micro-relief has been shown to be effective in reducing runoff and soil erosion and increasing plant establishment (See Section 10.1.2.4, Roughen Soil Surfaces).
Tillage — Tillage of the surface soil layers will leave the site in a roughened condition (See Section 10.1.2, Tillage). This practice has other beneficial effects on the soil besides leaving the surface in a roughened state.
5.6.8 Slope Length
Another factor influencing soil erosion and seed transport is the length of slope. As the slope lengthens, so does the potential for transport of sediment and seeds. On very long slopes, the erosive force begins as sheet erosion at the top of the disturbance and often turns into rill erosion at the bottom of the slope (Figure 5.53).
5.6.8.1 Slope Length — How to Assess
Slope length can be measured in the field or from road plans. The effects of slope length can be assessed by observing erosional features on existing disturbed areas. New or old disturbances can be used as references for critical slope lengths. As Figure 5.53 demonstrates, at some point downslope, erosional forces begin to cut rills into the surface soil. The distance downslope that this occurs can be considered the maximum length of slope for this site before severe erosion takes place. This distance will vary by the factors addressed in this section (soil cover, climate, slope gradient, surface strength, surface roughness, and infiltration rates).
5.6.8.2 Mitigating Measures For Long Slopes
Install Barriers — Fiber rolls, straw waddles, downed wood, hay bales, silt fences, or compost berms can be laid on the surface as obstructions or barriers to reduce slope length. To be effective, they must be placed in contact with the soil and perpendicular to the slope gradient. Straw waddles, hay bales, and silt fences must be trenched into the soil surface.
Create Benches — The creation of slope breaks can reduce slope length. These breaks can be benches, steps, or trenches cut into the slope. The reduced gradient at these breaks slows the velocity of overland flow and collects sediments.
If either practice is applied in a long continuous line, they must be constructed on contour. If they are not placed correctly along the contour, water can collect and move along the structure, much like a channel, and eventually spill onto the slope below, creating rills and gullies. (The exception to this is the construction of live pole drains to redirect water off-site for slope stability [See Section 10.3.3.4, Live Fascines].) When placed properly along the contour, slope distances are shortened and the structures collect sediments and create areas for plant growth. Plants that are grown above obstructions or near the bottom edge of benches can take advantage of water and sediments that collect during rainstorms. Native vegetation can be incorporated into many of these designs to take advantage of increased soil moisture and sediment accumulation (Figure 5.54). The distance that these structures are placed apart from each other should be no greater than the critical distance observed on the site.
5.7 Slope Stability
This discussion is directed to non-engineers to simplify and make accessible basic slope stability concepts that must be understood in developing revegetation strategies. It is by no means a substitute for professional engineering expertise. Technical references for slope stability are many (including Carson and Kirby 1972; Spangler and Handy 1973; Brunsden and Prior 1984; Denning and others 1994), to which we refer the reader for a comprehensive review of this subject. For a detailed evaluation of the role of vegetation in slope stability, the reader is referred to Gray and Leiser (1982).
Creating stable slopes is essential for establishing healthy plant communities, but the reverse is equally true — establishing native vegetation is critical for stabilizing slopes. This discussion comes from the latter perspective of how to create the most favorable environment for establishing vegetation and how this approach can be integrated into the overall strategy of slope stabilization. A vegetated slope adds stability to slopes by 1) holding the soil together through a network of root systems and 2) removing water from the soil, which is the primary driving force behind most landslides.
Slope stability is the resistance of natural or artificial slopes to fail through gravitational forces. The landforms resulting from slope failures are called landslides and they are described by their morphology, movement rates, patterns, and scale. This section will focus on two general types of landslides that are common to road cuts and fills — slumps and debris slides (Figure 5.55). Slumps typically occur on deep soils that are cohesive (rich in clays). They tend to be deep seated and slow moving. Viewed in profile, the failure occurs in a circular motion, resulting in a series of tilted blocks and circular cracks. Debris slides are shallow, fast moving landslides that form on non-cohesive soils (e.g., sandy, gravelly). These landslides occur on steep slopes.
Water input is the driving force behind most slope stability problems encountered in road building projects. It comes as rainstorms, snowmelt, and often as diverted surface water from road drainage. As water increases in a potentially unstable slope, the added weight of the water in the soil plus the increased pressure of water in the pores (pore water pressure) eventually exceeds the strength of the soil, and the slope fails (Figure 5.56).
Increased water to a slope is especially a problem where a restrictive layer (a layer of soil that limits water movement) is close to the soil surface. As water moves through the surface horizon and encounters a restrictive layer, the water saturates the pores of the horizon above it, increasing the water pressure in the soil pores. The saturated horizon becomes heavier as the pores fill, and eventually the slope fails under the added weight of water and the increased pore water pressure.
Figure 5.55 — Common landslides typically associated with road construction. Modified after Varnes (1978) and Bedrossiand (1983). |
![]() |
The faster water moves through soil, the less susceptible a slope is to failure. The measured rate at which water is transmitted through a soil mass is its permeability. When permeability is high, water quickly moves out of the soil pores, reducing the potential for increased pore water pressure or slope weight. When permeability is low, water slowly moves through soil and builds up in the soil pores. On gentle slopes, this is not a problem; as slope steepness increases, gravitational forces acting on the slope raise the potential for slope failure. Slope length is another factor important to water movement because the longer the slope, the greater the build up of water near the base of the slope. This phenomenon explains why many slope failures occur in the mid to lower portions of fill slopes.
Whether a slope fails or not is ultimately due to the strength of the soil. Soil strength is affected by the amount of clay present in the soil (the more clay, the greater the soil strength), how compacted the material is (the greater soil compaction, the more stable the soil), and the presence of roots (more roots are better). Often compacting soils takes priority over creating optimum soil for roots as methods for increasing soil strength. We suggest in the following discussion that the factors for increasing soil strength be considered together and integrated to produce the optimum stability, while at the same time creating the most favorable environment for native vegetation.
This section will discuss each of the following parameters as they relate to increasing slope stability through revegetation treatments:
- Permeability,
- Restrictive layers,
- Water input,
- Slope length,
- Slope gradient, and
- Soil strength.
5.7.1 Permeability
The rate at which a volume of water moves through soil material is its permeability (technically referred to as hydraulic conductivity). Soils that have moderate to high permeability rates tend to be more stable than those with low permeability rates. Where permeability is low, water fills the soil pores but does not drain quickly, adding additional weight to the slope and increasing the pore pressure. Both factors reduce the overall strength of slopes and increase the likelihood that slope movement will occur when other conditions are right.
Soils with large interconnecting pores have a higher permeability than soils with smaller pores that are less interconnecting. Soil textures that are well-graded (soils that have only one particle size) typically have a higher permeability than poorly-graded soils (soils having a range of particle sizes from clays to small gravels). For example, poorly graded granitic soils have low permeability rates because the different sized particles are neatly packed together, restricting the pathways for water flow. Well-graded soils, such as pure sands and gravels, have high permeability because pores are large and interconnecting. Compacted soils, on the other hand, often have low permeability rates because of the reduced or destroyed interconnecting macro-pores.
5.7.1.1 Permeability — How to Assess
Simple field tests, such as percolation tests, historically utilized in assessing septic leach fields, can be used for determining permeability rates. A small hole is excavated and water is poured to a specified depth. The time to drain the water from the pit is measured in inches per hour. These tests are run at different soil depths to determine if permeability rates change. Results from percolation tests are subject to great variability. Nevertheless, they can indicate the relative permeability of different soil types or different soil disturbances.
Engineering laboratory tests for determining permeability include the constant head permeameter for coarse-grain soils. In this test, a soil sample is placed in a cylinder at the same density as the soils in the field. Water is introduced and allowed to saturate the sample. A constant water elevation, or head, is maintained as the water flows through the soil. The volume of water passing through the sample is collected and this provides a direct measurement of the flow rate per unit of time. The test can be repeated at various densities to determine the corresponding permeability. The test can also be repeated for various additions of organic amendments and compaction levels to determine the effects of these treatments on permeability. Consult a soil lab for how to collect and submit samples for these tests. Where testing is not feasible, engineering and soil texts can give ranges for expected permeability based upon the soil gradation and classification. A field assessment can also give some indication of permeability rates. Subsoils or soils lacking organic matter that have a range of soil particle sizes, from clays through small gravels, have a propensity for low permeability rates, especially when they are compacted (See Section 5.3.3.1, Soil Structure — How to Assess).
5.7.1.2 Mitigating For Low Permeability
Tillage — Loosening compacted soil through tillage practices (See Section 10.1.2, Tillage) increases permeability by creating large fractures or pathways for water to flow. However, tilled soils often return to near-original permeabilities as the soils settle over time.
Organic Amendments — Long-fibered organic matter tilled deeply into the soil will increase the infiltration and permeability of the soil because larger, interconnecting pore spaces are created (See Section 10.1.5, Organic Matter Amendments). Several studies evaluating the incorporation of unscreened yard waste suggests that an optimum rate of organic matter additions for increasing infiltration and improving soil structure is approximately 25% compost to soil volume (Claassen 2006). In addition, incorporating organic matter can also increase slope stability because amended soils are lighter in weight than non-amended soils; mineral soils can weigh 10 to 20 times more than soils amended with 25% organic matter. The reduced soil weight lowers the driving forces that create unstable slopes. Additions of organic matter with high C:N ratios can limit the establishment of vegetation. Using composted organic matter will reduce the length of time that nitrogen is unavailable.
5.7.2 Restrictive Layer
A restrictive layer is any soil horizon or stratum (including unfractured bedrock) that has very low permeability. As water flows through a surface horizon with good permeability and encounters a restrictive layer, the rate of downward vertical water movement slows and water builds up in the pores of the upper layer. Since the permeability of the surface layer is higher than the restrictive layer, water moves laterally downslope above the contact. Slope failure occurs when the pores in the soils above the restrictive layer become saturated with water to a point where the pore water pressure and soil weight exceeds the soil strength. Failure occurs within the soils above the restrictive layer. The depth of slope failure depends on the thickness of the surface layer. On slopes where the restrictive layer is near the surface, slope failure, if it occurs, will be shallow (Figure 5.57). Where the contact is deeper, the soil movement will be more extensive. The types of landslides that occur with restrictive layers are debris slides.
5.7.2.1 Restrictive Layer — How to Assess
Restrictive layers in natural settings can be inferred by the presence of seeps and springs. These features occur most often at the point where a restrictive layer is intercepted or exposed to the surface. These can be intermittent features that are observed in the winter or spring that dry up in the fall or winter. The vegetation around permanent and temporary seeps and springs is typically composed of water-loving species, such as sedges (Carex spp.) and rushes (Juncus spp.). Figure 5.58 shows how a seep is created when a cut slope intersects a restrictive layer.
On construction sites, restrictive layers can be created by placing a loose soil over a highly compacted subsoil. These layers can be identified in the field by determining soil strength using a soil penetrometer or shovel to find compacted or dense soils (See Section 5.3.3, Soil Structure). This layer is then assessed for the approximate amounts of silt and clay using the field texturing method. Dense or compacted soil layers that are high in silts and clays are likely to be very restrictive to water movement. Field permeability tests can be used to determine the rates of water flow (See Section 5.7.1.1, Permeability — How to Assess). Often restrictive layers are not observed until after construction when they manifest themselves as intermittent seeps or wet areas in cut and fill slopes. Geotechnical investigation will determine if these features are due to shallow water movement associated with restrictive layers or interception of deeper subsurface water interception.
5.7.2.2 Mitigating For Restrictive Layers
Tillage — If restrictive layers are within several feet of the soil surface, site treatments that break up portions of this layer can increase the permeability and increase stability. Site treatments that accomplish this are bucket tillage, deep ripping, spading, and fill cut (benching and backfilling) which are discussed in Section 10.1.2, Tillage. The drawback to tillage is that it will reduce soil strength in the short term until roots occupy the soil and increase soil strength. Temporary irrigation systems have been installed on sites where quick establishment of grass cover during summer and fall months is essential for slope stabilization prior to winter precipitation (Hogan 2007).
Organic Amendments — Where possible, the incorporation of organic matter will help keep the restrictive layer from returning to its original soil density and allow more time for roots to become established (See Section 10.1.5, Organic Matter Amendments). As mentioned above, soil strength might be reduced until vegetation has become established, but the negative effects of reduced soil strength could be offset by the increased permeability and reduced soil weight of the organic amendments.
Live Pole Drains — Live pole drains are constructed to intercept water from seepage areas and remove it through a system of interconnecting willow bundles (which act as a drain) to more stable areas, such as draws, ditches, or other waterways (See Section 10.3.3.4, Live Fascines). The interception and flow of water encourages the establishment and growth of willow cuttings along the length of the live pole drain.
5.7.3 Water Input
Water, which is the driving force behind most landslides, comes through rainfall, groundwater flow, snow melt, or diverted from other areas through road drainage. Landslides often occur after a series of strong winter storms have delivered a high amount of rainfall over a short period of time. Under these conditions, soils have not had sufficient time to drain before the next storms arrive. When warm storms bring rain to slopes with snow pack (called "rain on snow events"), water from rain plus melting snows is very high, increasing the probability for the occurrence of landslides on potentially unstable sites. Landslides can occur where water from road ditches or other road features drain water onto marginally unstable slopes. Major site factors that affect water input are: 1) rainfall duration and intensity, 2) rain-on-snow events, and 3) road drainage.
5.7.3.1 Water Input — How to Assess
See discussions under Section 5.2, Water Input for how to assess water input.
5.7.3.2 Mitigating For High Water Input
Proper Surface Drainage — Increased water is the driving force behind slumps and debris slides. Designing proper water drainage is probably the most important measure to implement for slope stability (Gedney and Weber 1978). Where road water is inadvertently routed into potentially unstable areas, there is a greater potential for slope failure (Fredricksen and Harr 1981). Road projects are designed to move storm water away from or out of unstable slopes as quickly as possible through road and slope drainage structures.
Species Selection — Select species that have adapted to wet soils. These include sedges (Carex spp.), rushes (Juncus spp.), bulrushes (Scirpus spp.), willows (Salix spp.), cottonwoods (Populus spp.), and cedars (Thuja spp. and Chamaecyparis spp. ). Since each species has a unique way, or strategy, of modifying the moisture regime of a site, planting a mixture of species is a way of assuring that all strategies are represented on the site. For instance, willows establish quickly and can draw large quantities of moisture from the soil, but only when the willows have leaves. Cedars, on the other hand, are slower to establish, but longer lived. They can withdraw moisture from the soil during the winter months, unlike deciduous species. When they are well established, they will intercept large amounts of water in the crowns, preventing precipitation from reaching the soil. Trees have a great ability to significantly deplete moisture at considerable depths (Gray and Leiser 1982). Wetland species, such as rushes and sedges, unlike many trees and shrub species, are not limited by saturated soils. They thrive and draw down moisture where other species will perish.
Live Pole Drains — The live pole drain (Polster 1997) is a biotechnical engineering technique where continuous willow bundles (See Section 10.3.3.4, Live Fascines) are placed across a slope, much like an open drain, to redirect water to a more stable area (channels, draws, and so on). Where small slump failures have occurred during or after construction, live pole drains can be installed to increase slope drainage, add root strength, and remove soil moisture from the slide mass.
Figure 5.59 — Slumps are characterized by scarps, cracks, and benches. Water collects on the benches and in the cracks where it is transmitted into the slide mass, creating continued instability. Water can be removed through a series of hand-dug surface ditches. Using the cracks as guides for the location of ditches, they are filled in with soil and dug wide enough for a willow fascine (See Section 10.3.3.4, Live Fascines) to be placed in the bottom (A). Called "live pole drains" (Polster 1997), these structures not only quickly move surface water off the slide to more stable areas, but the willow cuttings in the fascines, encouraged by the presence of high soil moisture, grow into dense vegetation (B) that stabilize the slide through the deep rooting and dewatering. |
![]() |
5.7.4 Slope Length
Slope length is important in stability because the longer the slope, the more water can concentrate in the lower portion of the slope. Increased water will increase pore water pressure and soil weights, decreasing the stability of the mid to lower sections of long slopes. This is one reason why slumps are often observed in the mid to lower slope positions of longer fill slopes.
5.7.4.1 Slope Length — How to Assess
Slope length can be obtained from road plans or measured directly in the field using a tape.
5.7.4.2 Mitigating For Long Slopes
Live Pole Drains — Using live pole drains (discussed in the previous section), shortens the distance water is transmitted through the hill slope by intercepting surface and subsurface water in ditches at frequent slope intervals. Captured water is transmitted downslope through a system of continuous fascines to a stable channel (Figure 5.59).
Create Benches — Another method of reducing slope length can be the creation of a slope break. These are benches, steps, or trenches cut into the slope. The reduced gradient at these breaks slows the velocity of overland flow and collects sediments. However, unless the water is directed off the slope, total water input to the slope is not reduced.
5.7.5 Slope Gradient
As slope gradient increases, the destabilizing gravitational forces acting on the slope become greater. On a level surface, the gravitational force stabilizes the soil mass. As the slope gradient increases, the amount of force available to resist sliding along the failure surface decreases.
5.7.5.1 Slope Gradient — How to Assess
Assessing slope gradient is discussed in Section 5.6.6.1, Slope Gradient — How to Assess.
5.7.5.2 Mitigating For Steep Slopes
Mitigating measures for steep slopes are discussed in Section 5.6.6.2, Mitigating for Steep Slope Gradients.
5.7.6 Soil Strength
Soil strength (technically referred to as shear strength) is the characteristic of soil particles to resist downslope forces. The major physical factors contributing to increased soil strength are: 1) reduced porosity (compacted soils), 2) greater range of particle sizes (poorly graded soils), 3) greater angularity of the soil particles, 4) greater surface roughness, and 5) presence of silts and clays that add cohesive strength (Hall and others 1994).
The biological components contributing to increased soil strength are the: 1) matrix of roots that reinforce the surface horizon, 2) roots that anchor an unstable soil mantle to stable subsoils or rock, and 3) stems (e.g., trunks of trees) that add support to the soil immediately uphill (Hall and others 1994) (Figure 5.60). The physical factors, unfortunately, do not always support the biological factors. For example, porosity should be of particular interest to the revegetation specialist. From an engineering standpoint, low porosity soils have greater soil strength than soils with high porosity because soil particles are packed closer together and interlock. Reducing porosity is accomplished through various methods of compaction. From a vegetation standpoint, however, this practice is very limiting to plant establishment and vegetative growth (See Section 5.3.3, Soil Structure). Balancing the needs of creating a healthy soil for optimum vegetation while still maintaining slope stability until established vegetation adds root strength to the soil is a challenge to engineers and revegetation specialists. Creating soils with high porosity will decrease soil strength in the short term. However, if this practice increases the productivity of the site, the long-term result is often a net increase in soil strength (Gray and Leiser 1982).
The growth habits of native species can greatly influence slope stability because each has a unique rooting pattern and root tensile strength. For instance, grass roots are very fibrous and abundant in the surface horizon, adding surface stability when the grass cover is high. Grass and forb roots, however, are not as strong and do not penetrate as deeply into the soil as tree roots, and add little strength at deeper depths (Gray and Leiser 1982). The roots of shrub and tree species, on the other hand, are long and deep rooted, with relatively high tensile strength (Gray and Leiser 1982). The main advantage of these species is the long vertical roots (taproots) that can cross failure planes and bind the soil strata together.
5.7.6.1 Soil Strength — How to Assess
While the revegetation specialist will probably never perform an engineering test for soil strength, it is important to understand that there are routine assessment methods used for this determination. These tests are especially important to consider if the revegetation specialist is advising that soils on potentially unstable sites be deeply tilled or amended with organic matter, since these practices can reduce soil strength until vegetation is established.
A common method engineers use to estimate soil strength is to correlate soil classification (from sieve analysis and the characteristics of clay particles) with published literature values. Shear vanes or cone penetrometers are good methods to approximate the strength of fine-grained soils in the field. Published research is used to correlate field instrument readings with laboratory shear strength test results.
The triaxial shear test is a more precise laboratory method to determine shear strength of soils. In this test, a long cylinder of the soil is placed in a latex membrane and submerged in a clear plastic cylinder filled with water. A vertical pressure is applied to the cylinder at a slow rate until the soil sample shears. Very sensitive strain gauges measure the soil displacement, applied forces, and any pore water pressures that develop. Various water pressures are applied to the cylinder to simulate the confining pressure of soil depth (Brunsden and Prior 1984). This test can be used to determine the shear strength of soils that have been amended with organic materials.
5.7.6.2 Mitigating For Low Soil Strength
Biotechnical Engineering Techniques — Many biotechnical slope stabilization techniques use vegetative cuttings from willows or other easy-to-root species to structurally reinforce the soil. As these materials root, they add further stabilization to the slopes through interconnecting root systems and soil moisture withdrawal. These practices include stake planting, pole planting, joint planting, brush layers, and branch packing (See Section 10.3.3, Installing Cuttings).
Shrub and Tree Seedlings — On drier sites, where willow cuttings are less likely to survive and grow, shrub and tree seedlings can be used. While these species are slower growing, they usually have deeper root systems and persist longer once they are established. Grass and forb species can quickly establish on drier sites, but soil strength is limited to the surface of the soil profile where the roots are most abundant. On potentially unstable sites, grasses should be grown between shrub and tree seedlings to add soil strength to the surface soil while tree and shrub species become established. On dry sites, however, grasses must be excluded around seedlings or vegetative cuttings until they have become established. Because of the steep, shallow nature of many of these sites, planting seedlings is not always practical or successful. Hydroseeding or hand sowing, covered by a surface mulch that will protect the surface from erosion for several years while the shrubs become established, should be considered.
Soil Improvement — Improving soil productivity will thereby increase the density of roots and increase slope stability (Hall and others 1994). Mitigating measures that improve water storage, organic matter, and nutrients should, with time, increase slope stability. Some practices, such as soil tillage, reduce soil strength in the short-term. However, once plants have become established, more roots occupy the soil and slope stability is increased. Tillage should be integrated with practices that quickly reestablish vegetation to assure that slope stability is not compromised in the short term. On slopes where root strength is critical for stability in the first year after construction, irrigation might be considered in order to quickly establish a dense vegetation cover.
5.8 Weeds
Weeds are unwanted vegetation on a construction site or surrounding areas before, during or after revegetation. Roadsides can be major conduits for weeds, including invasive, noxious, introduced, and exotic species problematic not only for roadside ecology, but also for the general ecosystem health of surrounding lands and economic well-being of adjacent farmers, ranchers, gardeners, and other land managers. A poorly revegetated roadside can contribute to the spread of weeds and detract from environmental health. On the other hand, a good native revegetation plan can benefit surrounding environments. The best management will focus not on fighting weeds, but on establishing healthy, weed-resistant communities of desirable vegetation.
For the revegetation specialist, the term "weeds" denotes any species (usually non-native) that is limiting to successful revegetation with native plants due to increased competition. Weeds may be listed on federal, state, or local lists as noxious weeds or invasive species. The exact list of weed species will generally be determined on a project-by-project basis.
Categories of weeds include:
- Invasive,
- Noxious,
- Competing,
- Introduced, and
- Exotic.
The potential for weed species to become established after construction is a function of two site parameters:
- The growing environment. Are areas within the construction project favorable environments for weed establishment? These areas are often highly disturbed sites with bare soils.
- Weed sources. Are there existing weed populations and seed sources on the site prior to construction, or is there a potential that weeds can be brought into the site during construction? Sources of weeds are existing seed banks, infested adjacent lands, or transported from infested areas by vehicles.
Construction projects should consider the development of a weed management strategy that addresses how to control the plant environment and the plant source in the early stages of planning. Ideally, this strategy should be implemented 2-3 years prior to actual construction, continued through the construction phase, and up to 3 years post-construction. Depending on the severity of undesirable vegetation, additional long term treatments may be needed. Treatment of material sources and spoils areas should also be included in planning.
5.8.1 Growing Environment For Weeds
The strategy for keeping weeds out of a project area should focus ultimately on developing a growing environment that encourages healthy native plant communities over weedy species. The presence or introduction of weeds into an area does not necessarily mean that these species will become established or spread. There must first be an opening or space in the native plant community for the plants to grow. These conditions almost always occur in a disturbed area, in openings where native plants and soil have been removed or disrupted. Secondly, the disturbed environment must be favorable for the undesirable species to take hold and thrive. This requires that the soils and climate be optimal for the environmental needs of the species. Third, the weed species must have an advantage over native species. If the weeds are more resilient or robust in their growth habits in both adjacent lands and disturbed sites (that is, cuts and fills) then they are more likely to become established.
5.8.1.1 Growing Environment For Weeds — How to Assess
Each undesirable plant species has unique environmental needs which must be considered when assessing if a site environment is conducive to invasion by that species. The following are site factors to evaluate:
- Bare soil surface — Most weed seeds need bare soil to germinate and become established. Protocols for monitoring bare soil are presented in Chapter 12.
- Soil nitrogen — Many weeds out-compete native plants on soils that are high in nitrogen because they are more adapted to utilizing nitrogen during early plant establishment. Unless fertilizers are added to the site, soil nitrogen levels are typically low after construction.
- Light — Weeds tend to require full or partial sunlight to thrive (Penny and Neal 2003).
- Soil quality — The potential that a healthy native plant community can be established after disturbance reduces the possibility for weed invasion. Optimum native plant establishment is dependent on the condition of the soil and climate. A compacted site, lacking topsoil and organic matter, is poor for establishing a native plant community which would ultimately keep weeds from invading. Unlike perennial plants, the roots of most annual weed species occur in the surface layer (Jackson and others 1988; Claassen and others 1995) and do not require deeper soils to survive and grow. Annual species therefore will have an advantage over perennials on compacted soils or shallow soils.
Based on the assessment of these factors, risk maps can be developed showing: 1) the location of weed sources in or adjacent to the project, and 2) the optimum habitats for each weed species. The combination of these maps will show the high risk areas and can be used to develop a strategy for weed control.
5.8.1.2 Reducing or Eliminating Weed Growing Environments
Protect Uninfested Ground and Minimize Ground Disturbances — Reducing the footprint of the project results in less area being disturbed and exposed to possible weed invasion.
Develop Optimum Environments for Quick Native Plant Recovery — Developing mitigating measures that create an optimum environment for native plant recovery will limit the establishment and spread of weeds (Sheley 2005). Site factors that restrict downward root growth of native perennial plant roots (e.g., compacted soil) give the advantage to weed species. Treat seed-bed preparation as an investment.
Retain Shade — To reduce the vigor of undesirable plants, retain as much shade as possible.
Apply Mulch — A mulch can be applied to the surface of a disturbed soil to create a poor germination environment for weed seeds (See Section 10.1.3, Mulches). The mulch should have high void spaces (typical of long-fiber mulch materials) and low water-holding capacity. These characteristics are unfavorable for seed germination. The deeper the mulch, the greater the control of weed establishment. Depending on the weed species, 2 to 4 inches of mulch are required to effectively control the establishment of many weed species (Pellett and Heleba 1995; Ozores-Hampton 1998; Ozores-Hampton and others 2002; Penny and Neal 2003).
Oversow Native Seed Mix — Sowing native seeds at high rates will "flood" bare soil with the seeds of desirable species, reducing the "germination sites" where weed species can become established. The objective of this strategy is to fill the voids first with desirable species to crowd out unwanted species (See Section 10.3.1, Seeding).
Establish Desirable Non-Native Plants If Establishment of Natives Is Not Feasible — Due to surrounding site limitations, project parameters, timelines, or other circumstances, using locally adapted native plant materials may not always be possible. For example, if the project is surrounded largely or entirely by contiguous populations of cultivars, using locally adapted plant materials may largely be a waste. If the surrounding area is primarily native species, careful selection of cultivars should be considered. Many cultivar species, both native and introduced, have the potential to cross with their locally adapted native equivalent genera (See Section 6.4.1, Ensure Local Adaptation and Maintain Genetic Diversity).
Avoid Applying Nitrogen Fertilizers in First Year — High rates of nitrogen fertilizers can encourage the growth of weedy annuals at the expense of the establishment and growth patterns of native perennials (McLendon and Redente 1992; Claassen and Marler 1998), whereas perennial grasses have a competitive advantage at lower nitrogen levels (Welker and others 1991). Application rates of up to 108 lb/ac N(121 kg/ha N) have been shown to promote the establishment of introduced grasses over less competitive native grasses (DePuit and Coenenberg 1979). High rates of nitrogen fertilizers can also affect revegetation efforts by decreasing species richness and increasing the presence of non-native species (Munshower 1994; Wedin and Tilman 1996).When nitrogen fertilizer is applied at seeding, root systems of native perennials establish in the upper portion of the soil. The decrease in deep roots gives the annual weed species a competitive growth advantage (Claassen and others 1995). Limiting the amount of nitrogen fertilizer used the first year (during vegetation establishment) will help force the roots of perennials deeper into the soil where there is more moisture. The deeper root system increases the competitive advantage of the native perennials over the annual weeds. This strategy, however, does not preclude the need for fertilization. It might be that fertilizers are not applied until a year after sowing, when the native plants have become established (See Section 10.1.1, Fertilizers).
Apply High C:N Materials to High Nitrogen Sites — If soils are high in nitrogen, applying a high C:N material (e.g., wood products or hay) to the surface of the soil, or mixed into the soil, is another strategy to reduce the amount of available nitrogen for annual weed growth (REAP 1991; St John 1999). Over time, the organic material will break down and release nitrogen for uptake by perennials.
5.8.2 Weed Sources
Weed species come from sources already on the site, or are brought into the site from outside sources. They are typically propagated from transported seeds. In rare cases, they can arrive as live plants and plant parts (e.g., blackberry stems). Reproductive materials are brought in on construction equipment, cars and trucks, shoes and socks. The source of these materials are often sand, gravel, and rock used for road construction materials. All too often, weeds arrive during the revegetation efforts in contaminated mulches, topsoil, and uncertified seed sources.
5.8.2.1 Weed Sources — How to Assess
During the early phase of planning, vegetation surveys, noxious weed inventories (conducted during the environmental assessment), and topsoil surveys will locate and identify weeds. From these surveys, a noxious weed map can be developed which can then be used in the development of a weed control strategy for the project site. County weed boards and county and state road management staff may also be a source of information, as they track and treat weeds.
The backbone of a weed control strategy is a thorough understanding of the physiology and ecology of the weed species. The questions to answer for each potential species include:
- How do they propagate?
- How do they spread?
- What is their rate of spread?
- What are their growth habits during the year?
- How long do they survive?
- What is their life cycle?
- Are they annual, biennial, or perennial?
- Is there potential for an ecological progression to more aggressive species (e.g., cheatgrass infestations may give rise to more aggressive species such as knapweed)?
- What are the control methods and how effective are they?
- What are their environmental needs?
- What are their soil type preferences?
- What are their nitrogen needs?
- What sort of environment is needed for seed establishment (e.g., bare soil)?
- What are their climate preferences?
- Do they grow well in direct sunlight or do they prefer shade?
5.8.2.2 Preventing the Introduction of Weed Sources
Control of weeds falls into two categories — prevention and treatment. Preventing the introduction of a weed species is always the preferred strategy because it is easier and more economical to prevent the introduction of a weed species than to control or eliminate it once it has become established. If prevention fails, however, and weeds have become established, then treatment strategies should be implemented to contain and eliminate the problem.
Identify and Monitor Weed Populations — Locate weed populations early in the planning phases. Early detection and rapid response is critical to preventing weed infestation. Develop a systematic detection strategy. Identify and treat new patches before they spread (Sheley 2005).
Clean Equipment When Leaving Weed-Infested Sites — When equipment or vehicles have been used in an area where weed species are present, it is important to thoroughly wash the tires, wheel wells, and chassis to eliminate the possibility that weed seeds are spread when the equipment is transported to another site. Portable wash stations may be set up for treating newly arriving equipment.
Clean Hydroseeding Tanks, Range Drills, Other Seed Delivery Systems — Unless equipment is brand new, seed delivery systems have been used at other projects and can contain plant species that are not wanted on the next site. A thorough cleaning and inspection of this equipment is essential in eliminating the potential introduction of weeds. Hydroseeding tanks must be washed out and range drills air-blown before entering the project site.
Know the Quality of Seed Sources — Native grass and forb seed sources can contain weed seeds that were harvested along with the native seeds. The quantity of undesirable species present in propagated seed sources is dependent on the weed control practices and seed cleaning technology implemented by the seed producer. Because of the possibility of contamination of native seeds with weed seeds, seed testing must be conducted for all seeds that are purchased or procured. Purity tests will reveal the contaminants in a seedlot, including weed seeds, other plant seeds, and inert material. Testing is conducted through certified seed labs according to the Association of Seed Technologists Association (AOSTA) standards. Seed labs test for the presence of state-listed noxious weeds and other crop species. It is important to check state and federal lists to determine if any local weed species should be added to the testing list. Do not buy seeds with an undesirable or untested weed component.
Know Quality of Mulches — Buy mulch that is free of weed seeds. Hay and straw mulches are of special concern. Some states certify hay or straw as "weed-free." This means that the material is free of noxious weeds. It does not mean that the material is free of seeds, however. Some straw comes from the stubble left after a seed harvest of native grasses, which often includes unharvested seeds. These seeds are viable and will establish into plants when the straw is applied. If this is the origin of the straw, it is important to obtain the species and seed zone of the seed crop to determine if this material is appropriate for your project. A site visit prior to hay or straw cutting is a good way to assess if there are seeds of weed species in the material (Figure 5.63).
Know Quality of Compost Sources — Compost sources are not always free of weeds, especially if the materials were not composted properly. Compost temperatures must reach lethal temperatures and remain there long enough for plant seeds to be rendered nonviable. Fresh, moist compost piles, where temperatures are maintained between 140 to 160 °F for at least several days, will kill most pathogens and weed seeds (Epstein 1997; Daugovish and others 2006). When obtaining compost, make sure that the supplier complies with standards that meet the time-temperature requirements to ensure destruction of weed seeds. Testing and analysis information is available from the United States Composting Council (See Section 10.1.5, Organic Matter Amendments).
Inspect Gravel, Sand, and Rock Sources — Prior to acquiring road building materials such as sand, gravel, and rock, determine if this material comes from a source that is free of undesirable weeds. A plant survey of the source area will identify whether the material is suitable.
Inspect Haul Routes and Waste Areas — Treat or avoid infested areas.
Know the Quality of the Topsoil — The topsoil survey should identify areas of weeds (See Section 5.11.1, Survey Topsoil). These areas should be mapped out and avoided when the topsoil is salvaged. If the topsoil is purchased, the piles should be inspected prior to approval (Figure 5.64).
Do Not Store Sand, Gravel, Rock or Topsoil Near Weed Populations — Inspect the areas where topsoil, gravel, rock, sand, or other materials to be used on the construction project will be stockpiled. If there are weeds nearby, it is very likely that seeds will end up on these piles, especially if the piles are to remain there for over a year.
Restrict Livestock Grazing from Areas Infested by Weeds — Cattle can bring weed seeds from offsite where they have been grazing. Until native vegetation has become established, it is important to keep livestock out of the area.
5.8.2.3 Treating Weed Sources
If weeds are present on a project site prior to construction, or become established during or after construction, then treatments for control or eradication of these plants from the site must be assessed. The types of treatments fall into 4 categories (adapted from Massu 2005).
Manual — Hand-pulling weeds can be the easiest and quickest method of weed control. There are several methods or strategies for weeding. One strategy, the Bradley Method, prioritizes the areas to be weeded, starting in the areas with the least infestation (best stands of native plants) and proceeding to the worst. Once the weeded area is revegetated in native plants, weeding is continued into the more infested areas.
Mechanical — Mowing and cutting are mechanical treatments that can reduce the spread of seeds if they are done prior to the development of seed heads. Steaming and foaming are other mechanical treatments that can kill seeds and young plants.
Biological — Biological control agents exist for some weeds (Massu 2005). Information on biological controls can be found at http://www.aphis.usda.gov/ppq/weeds.
Chemical — Herbicides should only be used with the objective of establishing desired species. There are many effective herbicides on the market but not all are registered for commonly occurring weed species. When selecting an herbicide, it is important to find those products that are effective in controlling the target species yet have a minimal effect on non-target species. Using an appropriate application rate is an important factor for obtaining the optimum effectiveness of the herbicide. In addition, timing the application when desirable species are dormant could effectively protect the species. Treating areas with herbicides, prior to native seeding, requires care and a working understanding of the herbicides being used. To avoid toxic effects on establishing native vegetation after herbicide treatment, it is important to know the effectiveness, longevity, selectiveness, and application rates of the herbicides being considered for the project. Herbicides should only be used to temporarily open a site; the long-term goal is to establish vegetation to take the place of the weeds.
5.9 Pests
Many plant and animal species pass through or inhabit a newly revegetated site. When these organisms become problematic, they are called "pests." Part of good revegetation planning is to recognize the conditions where species might become pests on the project. This chapter focuses on mammals, insects, and diseases, that are the most common types of pests found on revegetation projects.
Many problems can be prevented through good design and management. For example, matching the appropriate plant species and stocktypes to the site reduces the potential of damage by insects and diseases. Where deer populations are high, planting unpalatable species reduces the risk that deer will over-browse newly planted vegetation or be encouraged to browse near the highway, creating a hazard to motorists. In many cases, the best investment is to preclude problems by protecting plants instead of attempting to control or destroy pests after they move in. However, not every sign of an animal or disease is cause for concern. Some loss due to herbivory or disease should be expected. Planning for some replanting after the first season is a good strategy to help establish native plants while allowing for some herbivory. During early establishment, when plants are most susceptible to life-threatening damage, treatment might be necessary. When this is the case, consider the system as a whole and select the least impactful, long-term treatment. For example, protection of plants with a physical barrier or a repellent spray might be better in the long-term than introducing a poison into the food chain that might also affect the animals that prey on the pests.
The following is a general discussion of mammals, insects, and diseases that can cause the most problems to reestablishing native vegetation. This is by no means a complete listing of issues or of mitigating measures. The reader is directed to other sources, such as Cleary and others (1988) and Helgerson and others (1992), for more a more thorough discussion of this topic.
Foraging mammals, such as deer, elk, gophers, and voles, may remove or destroy portions of the plant foliage or root systems. Feeding insects will weaken and, in some cases, kill establishing plants by feeding on roots, stems, and foliage. Diseases can be transported to the site on nursery stock and manifested under stressful transplanting conditions. Problems are usually encountered where the species were not appropriate for the site (e.g., dry species planted in a wetland), where there was not enough diversity in the species being planted, or on sites where soils were limiting (e.g., poorly drained soils). Unfortunately, the effects of pests on revegetation are not always easy to assess prior to implementation. Often the pest is not discovered until after revegetation has taken place.
5.9.1 Mammals
Mammals forage on the foliage, bark, and roots of young seedlings. In moderation, mammals will do minimal amount of damage to a revegetation project. Where herbivory is high, however, these animals can cause major damage to a newly planted site. The mammals of greatest concern to revegetation are the northern pocket gopher, deer, elk, and livestock.
5.9.1.1 Northern Pocket Gophers
Pocket gophers forage on all parts of the plants depending on the season of the year. Seedling stems are often completely debarked, thereby killing the tree (Figure 5.65). Gopher damaged seedlings lean to one side and are easily pulled from the soil (Helgerson and others 1992). Equally disruptive to plant establishment is the amount of soil that is brought to the surface during burrowing. Fresh gopher mounds can account for up to 18% of the soil surface (Laycock and Richardson 1975) and often bury newly establishing grasses, forbs, and seedlings. Gophers prefer soils that are low in rock fragments (<25%) and favor grasses and forbs over shrubs and trees. Succulent, perennial forbs, such as lupine (Lupinus spp.), buckwheat (Eriogonum spp.), and yarrow (Achillea spp.), are preferred over grasses (Bonar 1995). When present on a site, this small mammal can be an immense problem to establishing most plant species, especially those being planted from seedlings.
The potential of a revegetation project to become populated by gophers is a function of: 1) the presence of gophers in the adjoining lands, and 2) the favorability of the project site as gopher habitat. It should be assumed that if the project site borders areas populated by gophers, they will naturally move into the openings created by the road construction project. If the construction project is a poor habitat for gophers (e.g., rocky, steep, high slash), gophers will likely be less of a problem.
Prevention and Treatment — Leaving snags near gopher infested sites can help reduce populations by providing perches for raptors to prey on gophers. Physical barriers such as plastic netting have mixed results in reducing gopher damage. Another strategy is to create an unfavorable environment for gophers, including soils high in rock fragments, steep slopes, and sites with heavy slash (Bonar 1995). Planting seedlings at higher density or replanting seedlings that have been killed by gophers is another strategy. Since grasses and forbs are a preferred food source for gophers, planting shrubs and trees and covering the site with a deep mulch may discourage high populations.
Control of pocket gophers can be accomplished at various levels of effectiveness by trapping or baiting, encouraging predators, and protecting with netting or barriers. Trapping and toxic baiting appear to be the most widely used method of gopher control (Bonar 1995).
5.9.1.2 Deer and Elk
The new vegetative growth from tree and shrub seedlings is a preferred food source for deer and elk. Damage is observed as clean, sharp cuts to the branch or leader stems. Deer and elk typically leave scat and tracks on the sites where they browse. The scat is observed as scatterings or piles of large oval pellets. Trees that are annually browsed by deer or elk will remain stunted or low-growing for many years (Figure 5.66).
Figure 5.66 — The annual browsing of this Douglas-fir seedling by deer has created a seedling that is bushy and stunted with many buds. Frost damaged seedlings have a similar appearance. |
Prevention and Treatment — During the planning stages, consultation with the local wildlife and reforestation experts will help assess the potential for herbivory by deer and elk herds. Visits to areas adjacent to the project site can also give an indication as to the amount of damage that can be expected.
Planting non-palatable species in areas where high deer or elk populations are expected is a good preventive measure. Protecting seedlings from deer and elk browsing can be accomplished through fencing, application of animal repellents, installing plastic netting around the seedling, and planting large seedlings. Animal repellents are applied on or around the seedling with the objective of rendering the seedling unpalatable for browsing.
Fencing large planting areas to keep out elk and deer is used in places where herds are large. However, placing physical barriers, such as netting or tree shelters, around individual seedlings is the most common method of protecting seedlings. Rigid and non-rigid netting are commercially available products and are made from photo-degradable plastics that should break down after several years in the field (Figure 5.67). They are placed around the seedling after it is planted. Tree shelters will also protect seedlings during the early stages of establishment. Section 10.4 discusses these treatments in more depth.
5.9.1.3 Livestock
Damage to revegetation projects can be high in areas that are intensively grazed by cows or sheep. At high livestock populations, planted seedlings can be injured by rubbing and trampling. Seedlings are typically not browsed. Newly establishing native grass and forb cover can be harmed by the high-pressure hoof marks tearing up the new roots and surface soil, leaving the site exposed to non-native annual species.
Prevention and Treatment — Restricting the entry of livestock for several years after planting or sowing, or until native grasses and forbs have established, is the best prevention measure. This is typically accomplished by fencing the entire area being revegetated. Fencing is most effective when it is installed prior to establishing native vegetation. For this reason, having the fence installed as part of the road contract will assure that livestock is controlled prior to revegetation work. Working with the local USDA Forest Service or USDI Bureau of Land Management range conservationist will be necessary to assure that damage by livestock is kept to a minimum.
5.9.2 Insects
There are a variety of insects that can damage newly planted seedlings. These will be discussed briefly in this section. Since this is a specialized field, the reader is advised to consult technical or academic expertise if insect problems are found to be extensive on a revegetation project. Insect damage is grouped into four classes (Helgerson and others 1992), based on where insect feeding occurs:
- Sap-suckers — foliage,
- Root beetles — root system,
- Terminal feeders — terminal shoots, and
- Secondary bark beetles — stems.
It is very difficult during the planning stages to determine if insects will be a limiting factor. However, the local agency reforestation personnel should be consulted to determine which insects pose potential damage.
5.9.2.1 Insects — How to Assess
Damage to seedlings by insects is often overlooked because the injury usually occurs long before the seedling shows visible signs of stress. By this time, the insect has often left the scene, leaving only the telltale sign of activity. When dead or dying seedlings are discovered, it is important to systematically evaluate the seedling from the root system to the terminal bud for the presence of insects, insect damage, and disease (which will be discussed in the following section). If insects are found, individuals should be collected for later identification. It is important to look under the leaves for aphids and scrape the entire seedling with a sharp knife to observe boring and tunneling. Much of the damage caused by insects and disease occurs under the bark of the stems and roots themselves. A hand lens is helpful to detect microscopic damage. The following discusses some of the most prominent insects that damage conifer seedlings. Non-conifer species will have their own unique associated pests. Nevertheless, determining to which of the 4 classes the insect belongs is a start in making an appropriate assessment.
Sap-Suckers — Sap-suckers include the Cooley spruce gall adelgid (Adelges cooleyi) and the giant conifer aphids (Cinara spp.) which feed on succulent foliage of tree seedlings. While these can cause shoot deformity and foliage loss, aphids will normally not kill seedlings. The appearance of aphid ants (which cultivate aphid populations) on the leaves are indications that aphids are present.
Root Beetles — The root bark beetles (Hylastes nigrinus) and root-collar weevil (Steremnius carinatus) girdle the roots and stems of conifer seedlings and will weaken or kill newly planted seedlings. The damage can be mistaken for herbivory by small mammals, but the lack of teeth marks and the below-ground location of the damage are indications that the damage is caused by root beetles.
Terminal Feeders — The larvae of this group of insects feed on the terminal shoots of young conifers, killing much of the new growth. Continued annual attack by these insects can severely stunt conifer seedlings. The major insects include white pine weevil (Pissodes strobi [Peck]), ponderosa pine tip moth (Rhyacionia zozana), western pine shoot borer (Eucosma sonomana), and the cone worm (Dioryctria spp.).
Secondary Bark Beetles — The Douglas-fir engraver beetle (Scolytus unispinosus) attacks the stems of stressed seedlings, creating galleries under the bark that weaken or kill Douglas-fir seedlings.
5.9.2.2 Mitigating For Insects
Plant a Variety of Species — Insects are often host-specific, meaning they attack only one species. For this reason a preventative measure is to plant a variety of species. If an insect infestation occurs, it would not affect all the seedlings planted.
Plant Healthy Stock — Insects often attack weakened seedlings or seedlings under stress. Planting only healthy seedlings, appropriate to the site, reduces the potential damage by insects.
Create a Healthy Soil Environment — Seedlings grown on poor sites, or on sites outside of the species environmental ranges, will be placed under stress and become candidates for insect damage. Planting seedlings on optimal growing sites will produce healthy seedlings resistant to insect damage. Judicious use of fertilizers (that is, avoid overfertilization) is also important for pest prevention. For example, sucking insects often attack plants that have been over-fertilized with nitrogen.
Install Bud Caps — Some terminal feeders can be controlled through the use of bud caps which are placed over the terminal in the spring prior to shoot growth (Goheen 2005). Bud caps are materials made out of paper or fine cloth that temporarily cover the terminal and prevent adult insects from laying eggs on the bud.
5.9.3 Diseases
Diseases affect all species used in revegetation, but assessing and mitigating for individual species is normally not done on revegetation projects. If disease occurs, it is often the result of trying to establish plant species in the wrong environment. For instance, species adapted to dry environments will be susceptible to root diseases if planted in wet soils where root pathogens are present. Species that have evolved to wet soil conditions are less likely to be affected. While diseases might be present on a site, this does not necessarily mean that plants will be affected. Much like humans, plants are always surrounded by a variety of pathogens, but it is not until they are put under stress that they become more susceptible to infection. The resistance of a plant to disease is therefore dependent on its health.
Planting appropriate species, using genetically appropriate stock, purchasing high quality plant materials, improving the soil, and mitigating for climate extremes all play an essential role in creating healthy plants capable of resisting diseases. If diseases are found on seedlings after planting, often they came from the nursery. While diseases will weaken the seedlings from the nursery, it is unlikely that the diseases will spread through the soils or site and infect other plants. Since the revegetation environment is dissimilar to the nursery or agricultural environment, diseases from the nursery tend not to persist on the plant materials once they are installed. Plants that have a minor infection might recover when grown in the wild. Diseases that run rampant in grass seed beds at seed production facilities are less likely to infect grasses when they are seeded on revegetation projects because the growing environment in the wild is very different.
- Back to Top -
5.10 Human Interface
An often overlooked factor of revegetating roadsides is the unplanned effects humans can have on site recovery. The human interface is the disturbance resulting from human activities that limit plant establishment and growth. These potential disturbances must be considered very early in the planning stages to assure that they are integrated into the revegetation plan. Disturbances fall into two categories — road maintenance and recreation use. Roads must be maintained, but sometimes road maintenance activities negatively affect the establishment and growth of vegetation. Road corridors can also be sites of unplanned recreational use by off-road vehicles, which can leave a site compacted and devoid of vegetation.
5.10.1 Road Maintenance
Roads are periodically maintained for many reasons which include: 1) clearance for sight and safety, 2) weed control, 3) fire hazard reduction, 4) winter safety, 5) erosion control, and 6) utilities maintenance. Each of these activities affect plant growth in either a positive or negative way.
5.10.1.1 Road Maintenance — How to Assess Impacts on Revegetation
Road maintenance objectives should be understood at the beginning of the project. A meeting should be held with the agency that will be overseeing the maintenance of the road after construction to discuss how to integrate revegetation and road maintenance objectives. Questions or issues to resolve include:
- Will the ditches be cleaned out or bladed? If so, how far up the cut slope will this activity cause instability?
- Will herbicides be used (Figure 5.68)? If so, how far from the road shoulder? How often?
- How far away will trees be cut for "line of sight" and will this distance change on curves?
- What utilities are in the road corridor and will vegetation affect them?
- Are the roads treated with gravel during the winter for traction? If so, how much gravel is annually applied? How far is it swept into the road shoulder or road corridor? Are the gravels recovered by scraping the shoulders?
- Is fire an issue and will fire protection treatments include thinning of vegetation?
- What about salts or other non-herbicide contaminants?
This is a partial list, and every project will have its own set of questions. The more these issues can be addressed early in the planning stage, the more effective will be the revegetation plan.
5.10.1.2 Mitigating For Road Maintenance Impacts
Blading for Erosion Control — Ditchlines at the base of steep cut slopes are depositional areas for rock and soil that have moved down from the slopes above. The rock and soil may fill the ditches, disrupting the flow of water in the ditch and creating potential road drainage problems during storms. Blading is the removal of the material that has filled in the ditchline and is a normal maintenance procedure for erosive cut slopes. This operation not only removes plants that were established in the ditchline, but also destabilizes the surface slopes immediately above the ditch. This can affect the revegetation of the entire slope. Mitigating measures include engineering design that reduces slope gradients (See Section 5.6.6.2, Mitigating for Steep Slope Gradients) or changing the road drainage system so that cleaning of ditches is not necessary. If neither measure is possible, assess whether it is worth implementing revegetation treatments, since revegetating unstable slopes is usually not successful.
Herbicide for Vegetation Control — Contact the government agency responsible for controlling weeds or vegetation in the road corridor and discuss their strategy for weed control. In areas where vegetation must be maintained, signs can be issued to notify operators not to apply herbicides (Figure 5.69). Do not apply revegetation treatments to areas where herbicides will be used to control roadside vegetation.
Graveling for Winter Safety — In areas where gravels are applied to road surfaces for traction during snow or icy conditions, there will be a buildup of gravels on the sides of the road through sweeping of the road surface or snow-blowing. Vegetation that has been established in these areas is often completely covered with gravel when the snow melts. It is important to learn if the road maintenance agency will recover this gravel for reuse, or let it accumulate. If gravel is recovered, this area will be in constant state of disturbance. Determine the salvage distance and do not apply seeds in this zone. In areas where gravels are not salvaged, select the species that will survive and grow in this unique growing environment. One strategy is to select species that respond favorably to being covered by gravels. These include species such as manzanita and willows that root from their stems when covered by soil or gravel. Tap-rooted species, such as lupines, can take advantage of such conditions because they can access moisture deep in the gravel deposits (Figure 5.70). Examine shoulders of existing roads to identify species that have adapted to these conditions and use these species for revegetating these areas.
Figure 5.69 — Placing no-spray signs is a method of identifying areas to protect from herbicide application. |
Clearance for "Line of Sight" and Safety — Trees and shrubs are often thinned or removed in areas where line-of-sight is impeded. Good communication with the government agency responsible for maintaining the road during the planning phase will help identify those areas not suitable for shrub and tree species. Select grasses and forbs or low growing shrubs (<2 to 3 ft tall) for these areas.
Protection of Utilities — Do not plant trees under or near utility structures, such as power lines. Discuss the planting plans with the maintenance agency to find out if there are other utilities that need protection.
5.10.2 Recreational Use
The road corridor is often used for recreational purposes that can disturb established vegetation. This recreation is not usually sanctioned or intended, but it exists in certain areas nonetheless. Recreational disturbances include off-road vehicle travel, mountain bike use, trails to recreational sites, and Christmas tree cutting.
5.10.2.1 Recreational Use — How to Assess Impacts on Revegetation
The environmental assessment should identify the public's demand for these activities and how they will affect short and long term vegetation goals. Obliterated roads, for instance, are often desirable places for off-road vehicles because they are open and flat. Roads bordering recreation destinations, such as favorite fishing holes, will have demands for access trails or scenic views that the public does not want blocked by tall vegetation. Public scoping should identify these needs.
5.10.2.2 Mitigating For Recreation Impacts
There are several approaches to mitigation, most of which are forms of awareness, protection, and exclusion. Intruders can be excluded physically with barriers, such as ditches, fences, and large rocks. Before these measures are put into place, communication should be tried first. For example, a sign explaining native revegetation efforts may help make potential users aware that they should take their activities elsewhere. Local residents are often great sources for ideas on how to approach these problems; off-road vehicle clubs are another. Educating the public on the purpose for the revegetation treatments can go a long way towards protection. Short paragraphs in the local newspapers or on the FHWA website for each project will help. Using local contractors to implement the revegetation work brings ownership to the project and the people can be great sources for support in the community.
5.11 Inventory Site Resources
Most project sites contain resources that can be used to meet revegetation objectives. Identifying site resources early in the planning process is essential so that potential resources are not inadvertently wasted. The more you can work with the resources and assets of the site, the more cost-effective, efficient, and effective the revegetation efforts will be. Physical resources to inventory include topsoil, duff, and litter, parent materials, woody materials, plant materials (seeds, seedlings, and cuttings), large rocks, and water (seeps, springs, creeks). Intangibles should also be considered, such as community cooperation and the local knowledge base.
Figure 5.71 — Example of a form for collecting topsoil information. |
![]() |
5.11.1 Survey Topsoil
One of the most important site resources for revegetation is topsoil. If considered early in the planning process, topsoil can be salvaged and reapplied to disturbed sites after construction. This is one of the best ways of increasing productivity on a disturbed site (See Section 10.1.4, Topsoil).
Topsoil is inventoried early in the planning process to evaluate topsoil quality and depth; costs, removal, and storage feasibility; and any other associated costs. Topsoil recovery is an expensive operation requiring knowledge of soil attributes. For this reason, a thorough soil survey must be conducted on those locations that will be disturbed. An example of soil and site information commonly collected for topsoil recovery is shown in Figure 5.71. The road in this example is planned through undisturbed forested lands. Soils data is collected every 50 meters (at road stations) due to the variability of the soils in this area. Where soils are very uniform, distances between plots can be increased. Soil texture, rock fragments, and depth of the topsoil are measured in the field. At selected intervals, or on different soil types, a sample is collected for lab analysis.
During topsoil survey, other site attributes that could affect the quality of topsoil should be noted. The most obvious are the locations of all noxious weeds. These sites should be avoided during topsoil salvage operations since the reapplication of these soils would spread these species across the project area.
The outcome of the topsoil survey is a short report and map in the revegetation plan showing the areas to salvage topsoil and the depth of salvage. If there are great differences between topsoil depths to salvage, this should be shown on the map. The report should discuss the fertility of the topsoil, how it should be stored, and whether the duff and litter is removed and stored separately. Areas should also be identified where topsoil should not be collected. The most typical areas are those which support undesirable non-native vegetation.
5.11.2 Survey Duff and Litter
Duff and litter are the dead plant material that has accumulated on the surface of the soil. The level of decomposition differentiates litter from duff. Litter is the layer of recently fallen, undecomposed leaves, needles, and branches; duff (which occurs immediately below the litter layer) is litter that is decomposed beyond recognition. The duff layer is dark, light-weight organic layer that occurs under forest environment. It is a large reserve of nutrients and carbon, and has a high water-hold capacity. Litter and duff layers protect the soil from erosion by absorbing the energy of rainfall impact and reducing overland flow. The litter and duff layers combined can be very thick, ranging from 1 to 4 inches depending on the productivity and climate of the site.
Litter layers typically have viable seeds that have fallen from the overstory vegetation. Under the right conditions, these seeds will germinate. If collected, stored and reapplied correctly, this natural seed bank can be used as a seed source. See Section 10.1.3.11, Litter and Duff for discussion of methods for collection and application.
Litter and duff layers can be assessed at the same time that topsoil surveys are conducted. The measurement is simple; using a ruler at collection intervals, record the thickness of the litter and duff layers.
5.11.3 Subsoil and Parent Material
Certain subsoils and parent materials can be salvaged during road construction and used to produce manufactured topsoil (See Section 10.1.4.5, Manufactured Topsoil). Textures low in rock content, including sandy loams, silt loams, loam, and sandy clay loams, are often good materials for manufactured soils. These are often found in areas where the parent materials are derived from alluvial or windblown deposits. They include river sands, pumice, volcanic sands, and loess.
5.11.4 Woody Material
Woody material consists of live and dead plant materials that will be wasted in the construction of the road project. This material includes tree boles, root wads, bark, and branches. During clearing and grubbing, these materials are often concentrated in piles and burned. All of these materials can be chipped or mulched and used as surface mulches (See Section 10.1.3.7, Wood Fiber) or soil amendments (See Section 10.1.5, Organic Matter Amendments). Large wood can be used for biotechnical engineering structures, obstacles for erosion control or as down-woody material for site productivity. Using these materials for revegetation purposes will lower overall project costs.
5.11.5 Plant Materials
As discussed under Section 10.2, plant materials consist of seeds (See Section 10.2.1, Collecting Wild Seeds), plants (See Section 10.2.3, Collecting Wild Plants), and cuttings (See Section 10.2.2, Collecting Wild Cuttings). If collected and stored correctly, these materials can be used to revegetate disturbed sites. Surveys of the project site prior to construction will indicate location and abundance of plant materials.
5.12 Phase Two Summary And Next Steps
Planning Phase Two identifies site factors that limit revegetation, defines mitigating measures, and inventories site resources. The next phase is Phase Three, where vegetation will be assessed and analyzed in order to match plant materials and revegetation methods to the site attributes. In Phase Four, a comprehensive strategy is developed that integrates the components from each phase into the Revegetation Plan.